Zujin Zhao, State Key Laboratory of Luminescent Materials and Devices, Guangdong Provincial Key Laboratory of Luminescence from Molecular Aggregates, South China University of Technology, Guangzhou 510640, Guangdong, China. E-mail: mszjzhao@scut.edu.cn
Abstract
Blue emitters are highly desired in organic light-emitting diodes (OLEDs), but their electroluminescence efficiencies and roll-offs are always less than satisfactory. In this work, a triazine-based deep-blue emitter (2PhCzTRZ-Cz) is designed and synthesized. It prefers high thermal stability with a decomposition temperature of up to 543 °C, and possesses strong deep-blue photoluminescence. The doped OLEDs using 2PhCzTRZ-Cz as an emitter attain deep-blue lights at 418-424 nm, with high maximum external quantum efficiencies (ηexts) of 4.46-5.68%, maximum luminances of 2,820-7,400 cd m-2, CIEy values < 0.1 and small efficiency roll-offs. In addition, multiple-resonance thermally activated delayed fluorescence OLED by using 2PhCzTRZ-Cz as host realizes a high maximum ηext of 21.5%, significantly higher than those of the device based on traditional mCBP host (12.9%). These outstanding performances demonstrate the great potential of 2PhCzTRZ-Cz as an emitter and host for OLEDs.
Keywords
1. Introduction
In the past decades, organic light-emitting diodes (OLEDs) have attracted great attention due to their unique advantages in the display area, including lightweight, good flexibility, and ease of fabricating[1-5]. For traditional organic fluorescent materials, their internal quantum efficiency (IQE) is limited to 25%[6]. In trying to break out of this limitation, researchers paid a heavy price to develop new materials including triplet-triplet annihilation (TTA)[7,8] and thermally activated delayed fluorescence (TADF)[9]. Specifically, TADF materials can achieve almost 100% exciton utilization through reverse intersystem crossing (RISC) from the lowest triplet excited state (T1) to the lowest singlet state (S1), but they generally face severe roll-offs. Recently, hybridized local and charge transfer (HLCT) fluorescent materials have been developed to become an important organic material system for high performance OLEDs due to their "hot exciton" channel[10] that can convert high level triplet (Tn, n ≥ 2) to S1 through the high-lying RISC (hRISC) process. Although the development of OLEDs has solved the problem of exciton utilization, there are still many problems for blue materials, including unsatisfactory electroluminescence (EL) efficiencies and severe efficiency roll-offs[11-15].
Researchers have made tremendous efforts to address these issues by developing new blue materials. In 2019, Su and co-workers[16] adopted a simple strategy to extend the non-conjugated fragments and molecular lengths by introducing a tri-spiral donor to triphenyltriazine for alleviating the aggregation-caused emission quenching, and synthesized a triazine-based TADF material, which achieves 90% horizontal orientation dipole ratio (Θ//) and high ηext of 33.3% in doped and 20.0% in non-doped sky-blue OLEDs. In 2022, Wang et al.[17] reported a molecule, namely ICZ-TAZ, that simultaneously realized short-wavelength ultraviolet (UV) or near ultraviolet (NUV) emission while maintaining a narrow full width at half maximum (FWHM) value. They designed an ingenious synthetic method to achieve emitters with a coplanar structure, and the UV/NUV OLEDs based on ICZ-TAZ achieve ηext of 3.26% at 388 nm and 4.02% at 406 nm with narrow FWHM of 32 nm and 46 nm, respectively. In 2023, Wong and co-workers[18] proposed a design strategy by integrating asymmetric oxygen-boron-nitrogen (O-B-N) multi-resonance (MR) unit into traditional N-B-N MR molecules to form a rigid and extended O-B-N-B-N MR π-skeleton. Three deep blue MR materials (namely OBN, MBN, and ODBN) were synthesized, in which ODBN can achieve a high ηext of 24.15% accompanied by a deep blue emission with the corresponding CIEy coordinate below 0.1.
Herein, we introduced two 9-phenylcarbazoles to weaken the electronegativity of the acceptor core of triazine, and then carbazole as a weak donor is also used to connect the core through a phenyl bridge to weaken the charge transfer (CT) effect of the entire molecule, ensuring its deep-blue emission. Based on the above design, a new triazine-based fluorescence material (2PhCzTRZ-Cz) is synthesized, which has high thermal stability of 543 °C and good photoluminescence (PL) quantum yield (ΦPL) of 38%. The OLEDs employing 2PhCzTRZ-Cz as an emitter achieve high maximum ηext s of 4.46-5.68% with CIEy values < 0.1 and small efficiency roll-offs. More inspiringly, when employing 2PhCzTRZCz as the host, high-performance green multiple-resonance (MR) TADF OLED can be realized with a high maximum ηext of 21.5%, much superior to that of the device based on traditional mCBP host (12.9%).
2. Experimental Section
2.1 General information
All the chemicals and reagents were purchased from commercial sources and used as received without further purification. The final product was subjected to vacuum sublimation to further improve purity before photoluminescence (PL) and electroluminescence (EL) properties investigations.1H and13C NMR spectra were measured on a Bruker AV 400 or 500 spectrometer in CDCl3 or CD2Cl2 at room temperature. High-resolution mass spectra (HRMS) was recorded on an Agilent1290/Bruker maXis impact mass spectrometer. Thermal gravimetric analysis (TGA) data were collected from a TG209F1 under nitrogen protection at a heating rate of 10 K min-1. Differential scanning calorimetric (DSC) analysis were performed on a DSC 214 Polymer under dry nitrogen at a heating rate of 10 °C min-1. UV-vis absorption spectra were measured on a Shimadzu UV-2600 spectrophotometer. PL spectra were recorded on a Horiba Fluoromax-4 spectrofluorometer. PL quantum yields were measured using a Hamamatsu absolute PL quantum yield spectrometer C11347 Quantaurus_QY. Transient PL decay spectra were measured under vacuum atmosphere for the films, using Quantaurus-Tau fluorescence lifetime measurement system (C11367-03, Hamamatsu Photonics Co., Japan). Cyclic voltammogram was performed in dichloromethane or dimethylformamide containing 0.1 M tetrabutylammonium hexafluorophosphate with the scan rate of 50 mV s-1, using a platinum wire as the auxiliary electrode, a glass carbon disk as the working electrode and Ag/Ag+ as the reference electrode, standardized for the redox couple ferricenium/ferrocene (Fc/Fc+). The ground-state geometry was optimized using the density function theory (DFT)[19] method with B3LYP functional at the basis set level of 6-31G **, and the energy levels of excited-states were carried out using the time-dependent DFT (TD-DFT) method with B3LYP functional at the basis set level of def2tzvp.
2.2 OLED fabrication and characterization
The glass substrates precoated with a 90-nm layer of indium tin oxide (ITO) with a sheet resistance of 15~20 Ω per square were successively cleaned in ultrasonic bath of acetone, isopropanol, detergent and deionized water, respectively, taking 10 minutes for each step. Then, the substrates were totally dried in a 70 °C oven. Before the fabrication processes, in order to improve the hole injection ability of ITO, the substrates were treated by O2 plasma for 10 minutes. The vacuum-deposited OLEDs were fabricated under a pressure of < 5 × 10-4 Pa in the Fangsheng OMV-FS450 vacuum deposition system. Organic materials, LiF and Al were deposited at rates of 1~2 A s-1, 0.1 A s-1 and 5 A s-1, respectively. The devices B1-B3 had their luminance-voltage-current density and external quantum efficiency characterized with a dual-channel Keithley 2614B source meter and a PIN-25D silicon photodiode. The EL spectra were obtained via an Ocean Optics USB 2000+ spectrometer, along with a Keithley 2614B source meter. And devices H1 and H2 had their luminance-voltage-current density and external quantum efficiency characterized with a Keithley 2400 Source Meter and a PhotoResearch PR670 spectroradiometer, and EL spectra were characterized with a Keithley 2400 Source Meter and a PhotoResearch PR670 spectroradiometer. The effective emitting area of the devices was 9 mm2, determined by the overlap between anode and cathode. All the characterizations for EL efficiencies were conducted at room temperature in ambient conditions without any encapsulation.
2.3 Synthesis and characterization
3,3'-(6-(4-Fluorophenyl)-1,3,5-triazine-2,4-diyl)bis(9-phenyl-9H-carbazole) (3) : The mixture of (9-phenyl-9 H -carbazol-3-yl) boronic acid (1) (5.74 g, 20 mmol), 2,4-dichloro-6-(4-fluorophenyl)-1,3,5-triazine (2) (2.45 g, 10 mmol), K2 CO3 aqueous solution (2 mol L-1, 50 mL), toluene (100 mL) and ethanol (50 mL) was added into a three-neck round-bottom flask. After bubbling through nitrogen for 15 min, Pd(PPh3)4 (0.48 g, 0.50 mmol) was added into the solution and heated to 100 °C with stirring for 12 h under nitrogen atmosphere. The reaction mixture was washed with water (5 × 200 mL) and dried over Na2SO4. The solvent was removed by rotary evaporation and the crude product was purified by silica gel column chromatography using dichloromethane/petroleum ether (1:3, v/v) to give the product 3 as white solid (4.28 g, 65%).1H NMR (500 MHz, CDCl3) δ 9.60 (d, J = 1.6 Hz, 2H), 8.91-8.87 (m, 4H), 8.38 (d, J = 7.6 Hz, 2H), 7.68-7.62 (m, 8H), 7.55-7.38 (m, 10H), 7.32-7.27 (m, 2H).
3,3'-(6-(4-(9H-carbazol-9-yl)phenyl)-1,3,5-triazine-2,4-diyl)bis(9-phenyl-9H-carbazole) (2PhCzTRZ-Cz) : A 250 mL two-necked flask was charged with the powder of 3 (3.29 g, 5 mmol), Cz (920 mg, 5.5 mmol), sodium hydride (0.40 g, 10 mmol) under the protection of nitrogen. The flask was cooled in an ice-bath and anhydrous N, N-dimethylformamide (120 mL) was added to the flask and the mixture was stirred at 0 °C for 0.5 h, and then stirred at 130 °C for 24 h. After the reaction was completed, the mixture was cooled down to room temperature. The mixture was quenched with water and was vacuum filtered under reduced pressure. The crude product was purified by silica gel column chromatography using dichloromethane/petroleum ether (1:1, v/v) to obtain 2PhCzTRZ-Cz as white solid (2.13g, 53%).1H NMR (500 MHz, CD2Cl2) δ 9.70 (d, J = 1.5 Hz, 2H), 9.14 (m, 2H), 8.97 (m, 2H), 8.42 (m, 2H), 8.20 (m, 2H), 7.88 (m, 2H), 7.66 (m, 10H), 7.58 (m, 4H), 7.54 (m, 6H), 7.41 (m, 2H), 7.35 (m, 2H).13C NMR (125 MHz, CD2Cl2) δ 143.73, 141.71, 140.52, 137.18, 130.54, 130.03, 128.26, 127.90, 127.15, 127.06, 126.58, 126.49, 126.15, 123.68, 121.83, 120.73, 120.71, 120.33, 120.31, 110.16, 110.03, 109.70. HRMS (ESI, m/z) calcd. for C57H36N6 [M + H]+ : 805.3001; found: 805.3074.
3. Results and Discussion
3.1 Synthesis and characterization
The target molecule 2PhCzTRZ-Cz was facilely synthesized by the two-step reaction as shown in Scheme S1 in Supporting Information. Initially, the intermediate 3,3'-(6-(4-fluorophenyl)-1,3,5-triazine-2,4-diyl)bis(9-phenyl-9 H -carbazole) (3) was obtained via a Suzuki reaction of (9-phenyl-9 H -carbazol-3-yl) boronic (1) acid and 2,4-dichloro-6-(4-fluorophenyl)-1,3,5-triazine (2). Subsequently, carbazole was coupled with the intermediate (3) to furnish the final product. The chemical structure of 2PhCzTRZ-Cz was well confirmed by nuclear magnetic resonance and high-resolution mass spectrometry, with satisfactory results. 2PhCzTRZ-Cz is of brilliant thermal and morphological stabilities, as evidenced by a high decomposition temperature (corresponding to 5% loss of initial weight) of 543 °C and a high glass-transition temperature of 180 °C (Figure S1). According to the half-wave potentials in oxidation and reduction curves in cyclic voltammetry measurement[20-23], the highest occupied molecular orbital (HOMO) and the lowest unoccupied molecular orbital (LUMO) energy levels of 2PhCzTRZ-Cz were obtained to be -5.03 and -2.87 eV, respectively (Figure S2).
3.2 Electronic structure
Theoretical simulation was conducted by density functional theory (DFT) and time-dependent DFT for exploring the electronic information of 2PhCzTRZ-Cz. The electron cloud distributions of HOMO, LUMO, and energy levels of excited states are shown in Figure 1. The HOMO is mainly distributed on the carbazole donor that connected to the core through a phenyl. At the same time, the LUMO is primarily located on the central triazine acceptor with a wide-bound region extending to adjacent three phenyl rings. Such excessive overlap of HOMO and LUMO leads to a large theoretical energy splitting between S1 and T1 of 0.41 eV, which is unfavored for the appearance of delayed fluorescence. However, the calculated energy levels of high-lying triplet states (Tn, n = 4, 5, 6) are very close to the S1 energy level, which may open a potential "hot exciton" channel to effectively utilize triplet excitons through an hRISC process, thus breaking the theoretical limit of 25% for exciton utilization in traditional fluorescence.
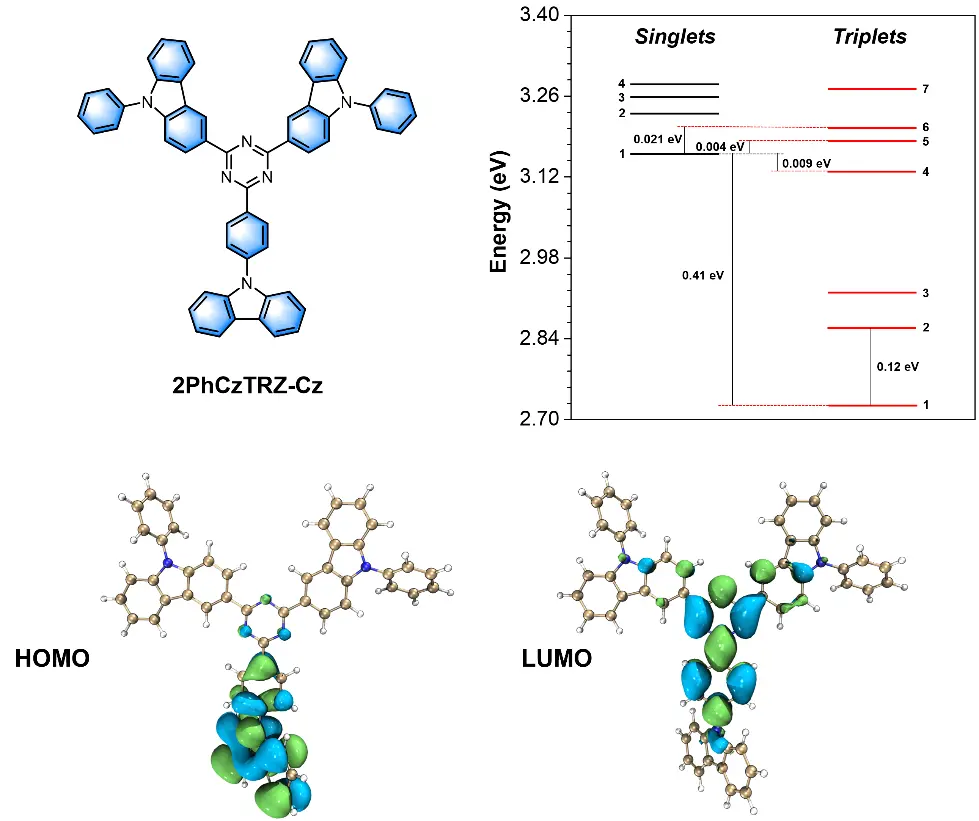
Figure 1. Molecular structure of 2PhCzTRZ-Cz, frontier molecular orbital distribution, and the calculated energy levels of excited states. HOMO: highest occupied molecular orbital; LUMO: lowest unoccupied molecular orbital.
3.3 Photophysical performances
The absorption and PL spectra of 2PhCzTRZ-Cz in dilute toluene solutions and PPF in dilute dichloromethane with a concentration of 10-5 M are displayed in Figure 2A and Figure S3. 2PhCzTRZ-Cz exhibits an absorption maximum at 322 nm, assigned to the π-π* transitions of the molecule. However, the discernible absorption peak from the CT transition cannot be found in the whole absorption curve, suggesting that the CT effect should be weak in 2PhCzTRZ-Cz. Owing to the weak CT effect, 2PhCzTRZ-Cz has a short-wavelength PL emission with a peak at 404 nm. The host of PPF shows an absorption maximum at 290 nm with a PL peak at 320 nm. To further evaluate the potentials of 2PhCzTRZ-Cz in OLEDs application, PL properties of 2PhCzTRZ-Cz in doped film were further investigated by using PPF (Table S1) as host with a doping concentration of 20 wt%. The doped film of 2PhCzTRZ-Cz shows a deep-blue PL peak at 433 nm (Figure 2B) with ΦPL of 38%, and a short PL lifetime of 11 ns with no obvious delayed component in the transient PL decay curve (Figure 2C). The main reason for the 19 nm red shift in the PL peak of dope film is that the PPF host has a large polarity. Meanwhile, such a short PL lifetime is insufficient to generate delayed fluorescence. According to the phosphorescence and fluorescence spectra of the doped film and toluene solutions measured at 77 K (Figure 2D and Figure S4), the ΔEST values are calculated to be as large as 0.41 eV and 0.45 eV respectively. Such large ΔEST values are unfavored for up-conversion of T1 to S1. Therefore, 2PhCzTRZ-Cz hasn't TADF characteristics. All the key photophysical and electrochemical properties of 2PhCzTRZ-Cz are summarized in Table 1.
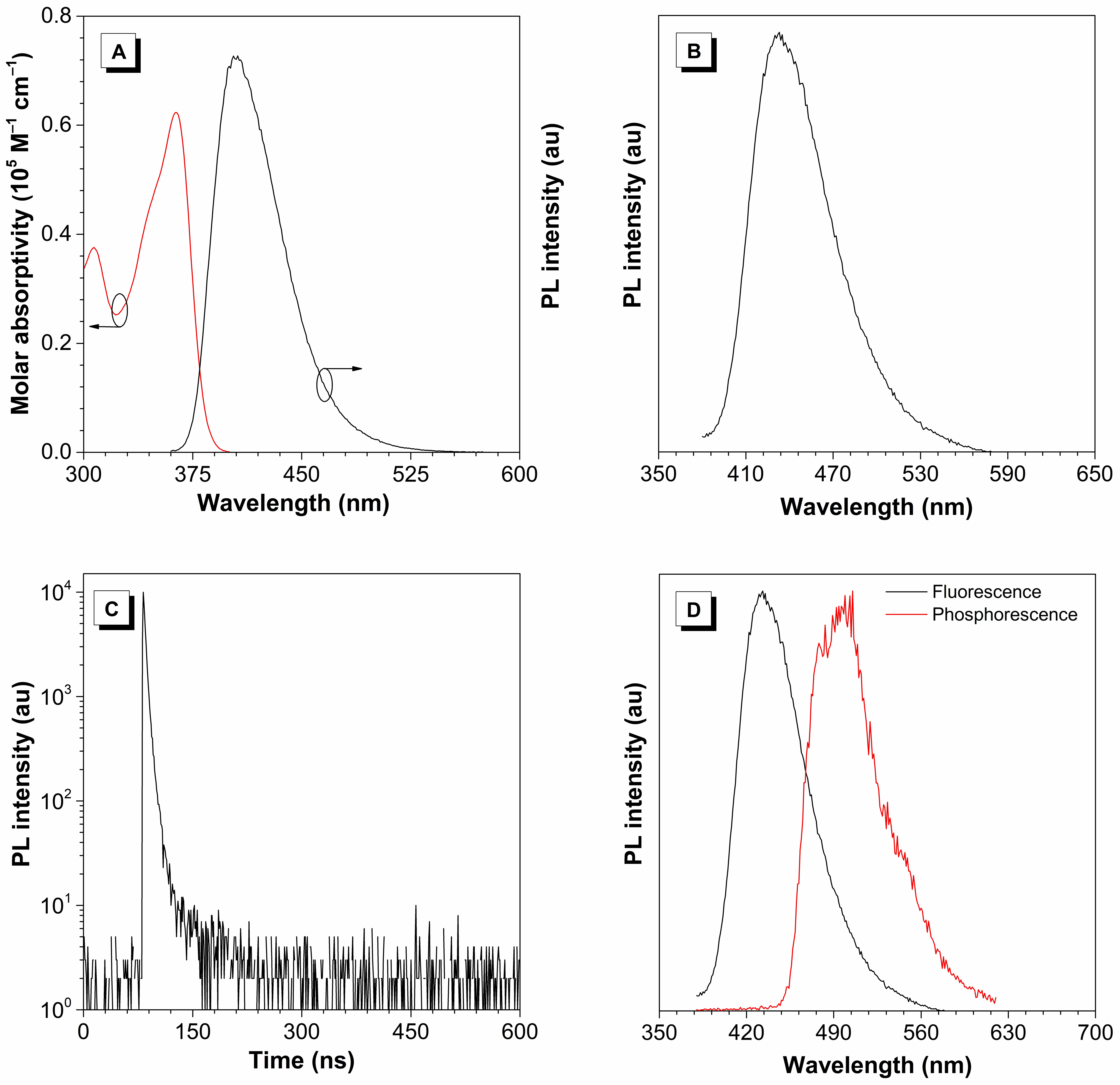
Figure 2. (A) Absorption and PL spectra of 2PhCzTRZ-Cz in dilute toluene solution (10-5 M); (B) PL and (C) transient PL decay spectra of the doped film (20 wt% 2PhCzTRZ-Cz: PPF); (D) Fluorescence and phosphorescence spectra of the doped film (20 wt% 2PhCzTRZ-Cz: PPF) measured at 77 K (delayed 20 ms). PL: photoluminescence.
Molecule | λabsa) [nm] | λemb) Tol/DF [nm] | ΦPLc) [%] | Τd) [ns] | HOMO/LUMOe) [eV] | ∆ ESTf) Tol/DF [eV] | Tdg) [°C] | Tgh) [°C] |
2PhCzTRZ-Cz | 322 | 404/433 | 38 | 11 | -5.03/-2.87 | 0.45/0.41 | 543 | 180 |
a) Measured in oxygen-free toluene solution (10-5 M) at room temperature;b) PL peak in Tol solution and DF in PPF (20 wt%) at room temperature;c) Absolute PL quantum yield determined by a calibrated integrating sphere under nitrogen at room temperature;d) Fluorescence lifetime;e) Cyclic voltammogram of 2PhCzTRZ-Cz measured in dichloromethane (HOMO) and dimethylformamide (LUMO);f) Doped film and tol solution measured at 77K;g) TGA andh) DSC curves of 2PhCzTRZ-Cz, measured under nitrogen at a scan rate of 10 °C s-1. PL: photoluminescence; HOMO: highest occupied molecular orbital; LUMO: lowest unoccupied molecular orbital; Tol: toluene; DF: doped film; TGA: thermogravimetric analysis; DSC: differential scanning calorimeter.
3.4 Electroluminescence
3.4.1 Blue fluorescent OLEDs
The OLED devices were fabricated by using the doped films of 2PhCzTRZ-Cz in PPF host with different doping concentrations as emitting layers (EMLs). The configuration of the devices is ITO/HATCN (6.5 nm)/TAPC (30 nm)/TCTA (5 nm)/ mCP (5 nm)/EMLs (20 nm)/PPF (5 nm)/TmPyPB (30 nm)/LiF (1 nm)/Al (EMLs are 10, 20, 30, 40, 60, 80, and 100 wt% 2PhCzTRZ-Cz: PPF for device B1, B2, B3, B4, B5, B6, and B7). Device configuration, energy diagram, and functional layers for the vacuum-deposited OLEDs are displayed in Figure 3A, Figure 3B, and Table S1. The EL parameters are summarized in Table 2, and the EL characteristics curves are depicted in Figure 3C, Figure 3D and Figure 3E. The B1-B7 devices radiate deep-blue light with EL peaks in the range of 418-424 nm and exhibit high maximum luminance (L) ranging from 2,820 to 9,150 cd m-2. Among these devices, B1 possesses the best EL efficiencies with maximum current efficiency (ηC), maximum power efficiency (ηP), and maximum ηext of 2.79 cd A-1, 2.58 lm W-1, and 5.68%, respectively, which is among the state-of-the-art deep-blue fluorescent OLEDs. The CIEy values of all devices are less than 0.1, confirming their pure deep-blue light features. More inspiringly, the efficiency roll-offs of B1-B7 from the maximum value to that at 1,000 cd m-2 are calculated to be 54.8%, 32.3%, 17%, 14.8%, 1.3%, 0.2%, and 0% respectively, indicating a trend of gradually decreased roll-offs with increased guest concentration, and the non-doped device has smallest roll-offs at 1,000 cd m-2. Compared to some recently reported deep blue molecules (Table S2), 2PhCzTRZ-Cz exhibits more satisfactory advantages in EL emission and maximum ηext. Considering the excellent EL performance, 2PhCzTRZ-Cz should theoretically have a high horizontal dipole orientation tendency. Therefore, the horizontal dipole ratio (Θ//) is measured by the angle-dependent p-polarization PL method. As depicted in Figure S5, 2PhCzTRZ-Cz possesses a large Θ// of 81.4%, which contributes to the high ηext over 5%.
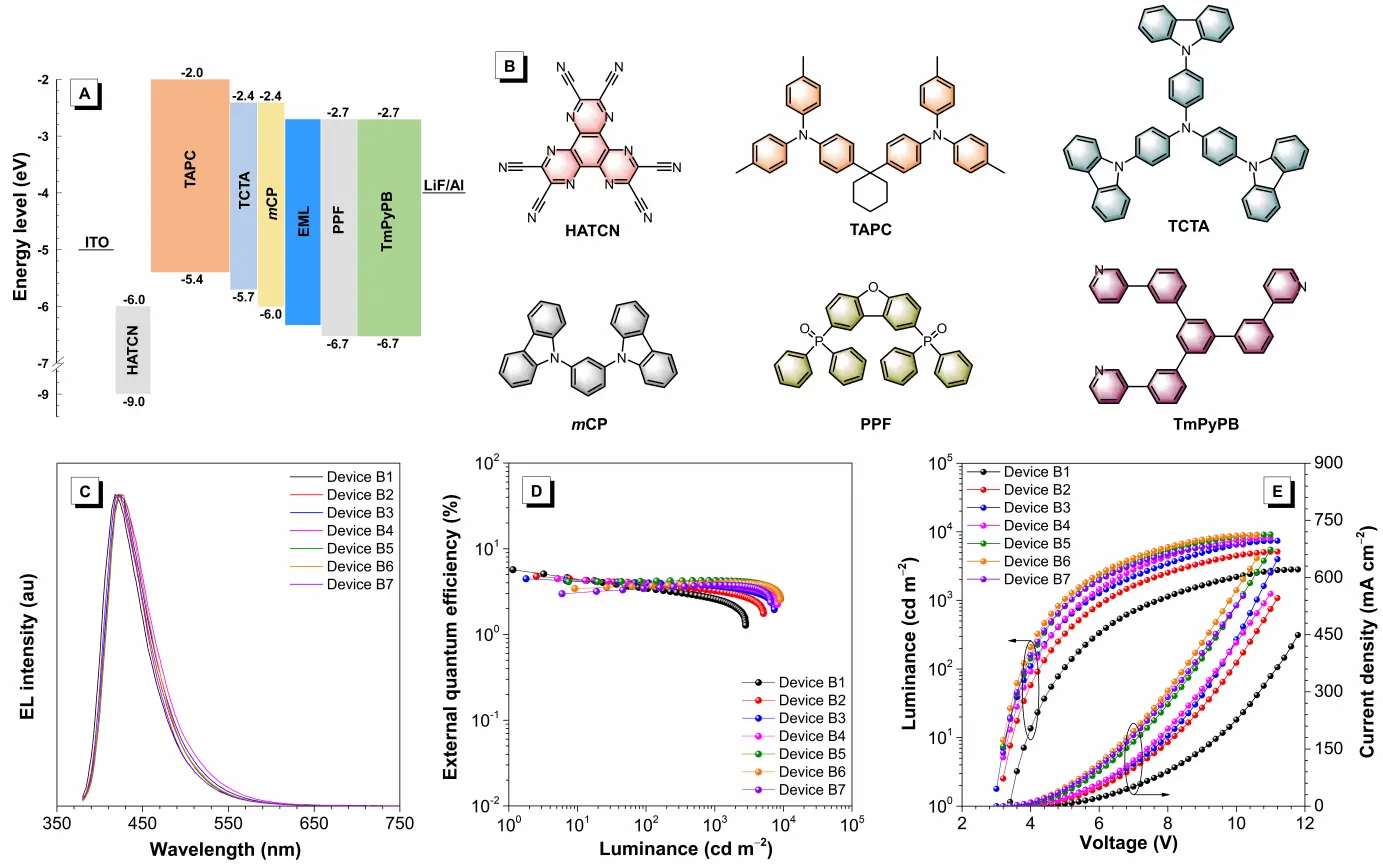
Figure 3. (A) Configuration and energy diagram, and (B) molecular structures of the functional materials of the deep-blue doped OLEDs based on 2PhCzTRZ-Cz; (C) EL spectra measured at 5 V; (D) external quantum efficiency curves with the change of luminance and (E) luminance and current density curves with the change of voltage of the blue OLEDs. OLEDs: organic light-emitting diodes; EL: electroluminescence.
Devicea) | λEL (nm) | Von (V) | Lmax (cd m-2) | ηC (cd A−1) | ηP (lm W-1) | ηext (%) | CIE (x, y) |
maximum value/at 1000 cd m-2 | |||||||
B1 | 418 | 3.3 | 2820 | 2.79/1.26 | 2.58/0.51 | 5.68/2.57 | (0.16, 0.07) |
B2 | 422 | 3.1 | 5160 | 2.56/1.74 | 2.51/0.88 | 4.77/3.23 | (0.16, 0.07) |
B3 | 424 | 2.9 | 7400 | 2.61/2.17 | 2.74/1.22 | 4.46/3.70 | (0.16, 0.08) |
B4 | 424 | 3.1 | 8210 | 2.94/2.50 | 2.90/1.40 | 4.45/3.79 | (0.16, 0.09) |
B5 | 424 | 3.1 | 9150 | 2.26/2.26 | 2.15/1.36 | 4.25/4.24 | (0.16, 0.07) |
B6 | 424 | 3.1 | 8970 | 2.16/2.14 | 1.82/1.34 | 3.99/3.94 | (0.16, 0.07) |
B7 | 424 | 3.1 | 6540 | 1.89/1.89 | 1.47/1.14 | 3.76/3.76 | (0.16, 0.07) |
λEL = electroluminescence peak at 5 V; Von = turn-on voltage at 1 cd m-2 ; Lmax = maximum luminance; ηC / ηP / ηext = current efficiency/power efficiency/external quantum efficiency; CIE = Commission Internationale de l'Eclairage coordinates at 1000 cd m-2 ;a) : abbreviations; EL: electroluminescence; OLEDs: organic light-emitting diodes.
3.4.2 Carrier mobility
Further, to detect the ability of this molecule as the host, we fabricated the single carrier devices. The hole-only and electron-only devices based on the structures of ITO/TAPC (10 nm)/2PhCzTRZ-Cz (80 nm)/TAPC (10 nm)/Al (120 nm) and ITO/TmPyPB (10 nm)/2PhCzTRZ-Cz (80 nm)/TmPyPB (10 nm)/LiF (1 nm)/Al (120 nm) were fabricated. Typical ohmic characteristics were observed at a low voltage based on the current density-voltage (J-V) curves. The space-chargelimited current (SCLC) property can be estimated using Mott-Gurney equation (1)[24]:
Where ε0 represents the vacuum permittivity (ε0 = 8.85 × 10-14 C V-1 cm-1), εr stands for the relative dielectric constant, which is considered to be 3.0 for organic semiconductor, E is the strength of an electric field, and L means the thickness of the neat film of an emitter. Apparently, from Figure 4, this molecule exhibits better electron transfer performance than hole transfer performance, with electron mobility three orders of magnitude higher than hole mobility. The fast electron transfer ability is offered by the core of triazine, while the hole transfer ability is mainly provided by the three carbazole groups.
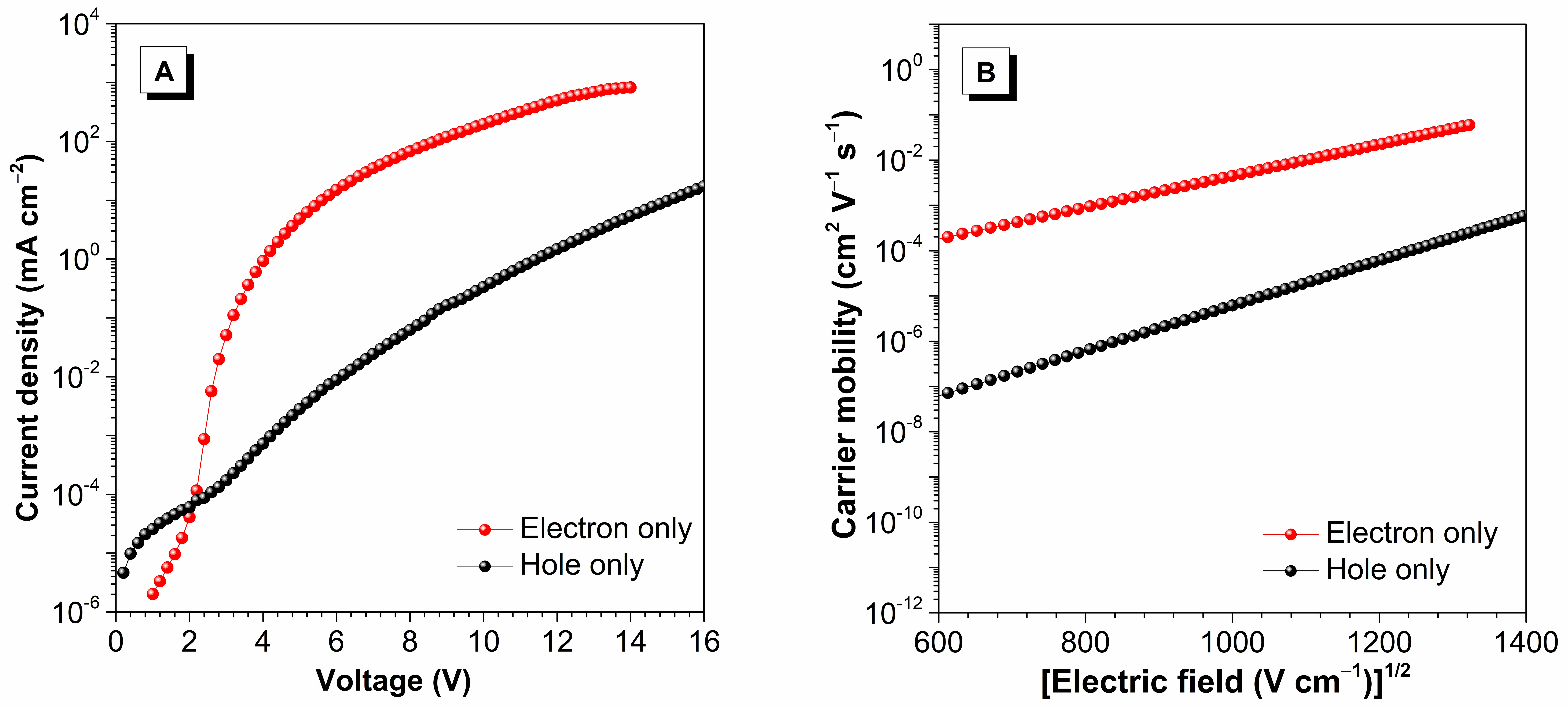
Figure 4. (A) Plots of current density-voltage and (B) plots of electric field-dependent mobilities (μ) of single carrier devices.
3.4.3 MR-TADF OLEDs employing 2PhCzTRZ-Cz as the host
To verify the ability of the molecule as the host, we selected a green MR-TADF molecule (BN2, Figure 5B) as the guest, 2PhCzTRZ-Cz was used as a host for device characterization. For comparison, the traditional host of mCBP was also used to prepare the device. The structure of the devices is ITO/HATCN (5 nm)/TAPC (30 nm)/TCTA (5 nm)/ mCP (5 nm)/EMLs (20 nm)/PPF (5 nm)/TPBi (50 nm)/LiF (1 nm)/Al (EMLs is 3 wt% BN2: 2PhCzTRZ-Cz for device H1 and EMLs is 3 wt% BN2: m CBP for device H2). The obtained device H1 has a lower turn-on voltage of 3.4 eV than device H1 (3.7 eV), indicative of more efficient injection and balanced transport of the charge carriers. Device H2 exhibits green emission peaking at 544 nm with a small FWHM of 42 nm and a maximum ηext of 12.9% (Figure 5 and Table 3). When employing 2PhCzTRZ-Cz as the host, the device H1 radiates green light with an EL peak at 544 nm and FWHM of 41 nm, which is coincident with those of the device H2. Very encouragingly, device H1 provides excellent EL performance with maximum L, ηC, ηP and ηext of 49,690 cd m-2, 89.7 cd A-1, 79.6 lm W-1, and 21.5%, respectively, significantly higher than those of the device based on mCBP host (17,440 cd m-2, 54.3 cd A-1, 41.2 lm W-1 and 12.9%). The significant improvement of EL performance by using 2PhCzTRZ-Cz as the host may mainly be due to the 2PhCzTRZ-Cz having better electron transport and result in more balanced carrier transport in the EML[25,26]. In addition, using the host with high Θ// can also induce the guest to have higher Θ//, which is contributed to the better EL efficiency[27]. Last, to ensure the reliability of the device data, both devices B1 and H1 were repeated by 4 times, and similar results were obtained (Figure S6), indicative of high repeatability.
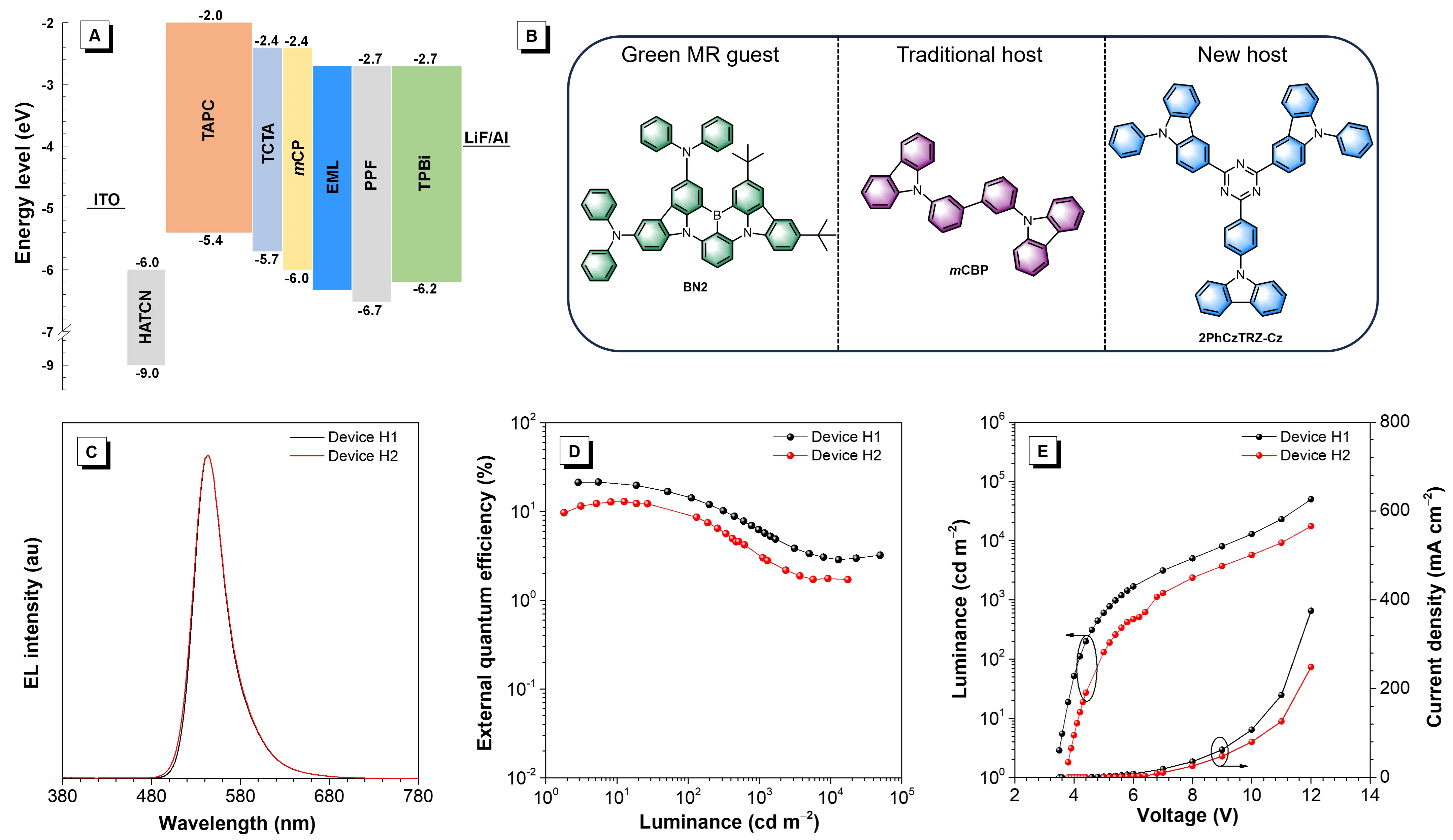
Figure 5. (A) Configuration and energy diagram; (B) Molecule structure of BN2, mCBP, and 2PhCzTRZ-Cz; (C) EL spectra measured at 5 V; (D) external quantum efficiency curves with the change of luminance; (E) luminance and current density curves with the change of voltage of the green OLEDs. OLEDs: organic light-emitting diodes; EL: electroluminescence.
Devicea) | λEL (nm) | Von (V) | Lmax (cd m-2) | ηC (cd A-1) | ηP (lm W-1) | ηext (%) | CIE (x, y) |
maximum value/at 1000 cd m-2 | |||||||
H1 | 544 | 3.4 | 49690 | 89.7/26.2 | 79.6/15.2 | 21.5/6.3 | (0.35, 0.64) |
B2 | 544 | 3.7 | 17440 | 54.3/12.6 | 41.2/5.8 | 12.9/3.0 | (0.34, 0.64) |
λEL = electroluminescence peak at 5 V; Von = turn-on voltage at 1 cd m-2 ; Lmax = maximum luminance; ηC / ηP / ηext = current efficiency/power efficiency/external quantum efficiency; CIE = Commission Internationale de l'Eclairage coordinates at 1000 cd m-2 ;a): abbreviations; EL: electroluminescence; OLEDs: organic light-emitting diodes.
4. Conclusion
In conclusion, an efficient deep-blue fluorescent molecule (2PhCzTRZ-Cz) comprised of triazine acceptor and carbazole donor is successfully developed. 2PhCzTRZ-Cz exhibits high thermal stability and possesses a deep-blue PL peak at 433 nm with ΦPL of 38% in doped film. Efficient pure deep-blue OLEDs (CIEy < 0.1) are fabricated with 2PhCzTRZ-Cz serving as emitter, providing excellent EL performances with maximum ηext of 3.76-5.68% at 418-424 nm and 0% roll-offs at 1,000 cd m-2 for the non-doped device, being among the state-of-the-art deep-blue fluorescent OLEDs. Especially, when 2PhCzTRZ-Cz serves as the host for MR-TADF guest (BN2), high-performance green narrowband OLED can be realized with a maximum ηext of 21.5% and small FWHM of 41 nm. These results demonstrate that 2PhCzTRZ-Cz is capable of both emitter and host for the fabrication of high-performance OLEDs.
Supplementary materials
The supplementary material for this article is available at: Supplementary materials.
Authors contribution
Zhao Z: Conceived and designed the experiments, wrote and revised the paper.
Tang BZ: Conceived and designed the experiments.
Guo T, Liu Z: Performed the experiments.
Jiang R: Wrote and revised the paper.
All authors approved the final version of the manuscript.
Conflicts of interest
The authors declare no conflict of interest.
Ethical approval and consent
Not applicable.
Availability of data and materials
The data and materials could be obtained from the corresponding authors upon request.
Funding
This work was financially supported by the National Natural Science Foundation of China (U23A20594 and 22375066), the Guangdong Basic and Applied Basic Research Foundation (2023B1515040003, 2022A1515010315 and 2021A1515110826) and Guangzhou Science and Technology Planning Project (202201010439).
Copyright
© The Author(s) 2025.
References
-
1. Tang CW, VanSlyke SA. Organic electroluminescent diodes. Appl Phys Lett. 1987;51(12):913-915.
[DOI] -
2. Rogers JA, Someya T, Huang Y. Materials and mechanics for stretchable electronics. Science. 2010;327(5973):1603-1607.
[DOI] -
3. Hwang J, Nagaraju P, Cho MJ, Choi DH. Aggregation-induced emission luminogens for organic light-emitting diodes with a single-component emitting layer. Aggregate. 2023;4(1):e199.
[DOI] -
4. Shao S, Wang L. Through-space charge transfer polymers for solution-processed organic light-emitting diodes. Aggregate. 2020;1(1):45-56.
[DOI] -
5. Yang G, Ran Y, Wu Y, Chen M, Bin Z, You J. Endowing imidazole derivatives with thermally activated delayed fluorescence and aggregation-induced emission properties for highly efficient non-doped organic light-emitting diodes. Aggregate. 2022;3(2):e127.
[DOI] -
6. Baldo MA, Lamansky S, Burrows PE, Thompson ME, Forrest SR. Very high-efficiency green organic light-emitting devices based on electrophosphorescence. Appl Phys Lett. 1999;75(1):4-6.
[DOI] -
7. Yanai N, Kimizuka N. New triplet sensitization routes for photon upconversion: thermally activated delayed fluorescence molecules, inorganic nanocrystals, and singlet-to-triplet absorption. Acc Chem Res. 2017;50(10):2487-2495.
[DOI] -
8. Jiang H, Tao P, Wong WY. Recent advances in triplet-triplet annihilation-based materials and their applications in electroluminescence. ACS Mater Lett. 2023;5(3):822-845.
[DOI] -
9. Uoyama H, Goushi K, Shizu K, Nomura H, Adachi C. Highly efficient organic light-emitting diodes from delayed fluorescence. Nature. 2012;492(7428):234-238.
[DOI] -
10. Hu D, Yao L, Yang B, Ma Y. Reverse intersystem crossing from upper triplet levels to excited singlet: a 'hot excition' path for organic light-emitting diodes. Phil Trans R Soc A. 2015;373(2044):20140318.
[DOI] -
11. Chan CY, Cui LS, Kim JU, Nakanotani H, Adachi C. Rational molecular design for deep-blue thermally activated delayed fluorescence emitters. Adv Funct Mater. 2018;28(11):1706023.
[DOI] -
12. Zhang D, Cai M, Zhang Y, Zhang D, Duan L. Sterically shielded blue thermally activated delayed fluorescence emitters with improved efficiency and stability. Mater Horiz. 2016;3(2):145-151.
[DOI] -
13. Kim M, Jeon SK, Hwang SH, Lee JY. Stable blue thermally activated delayed fluorescent organic light-emitting diodes with three times longer lifetime than phosphorescent organic light-emitting diodes. Adv Mater. 2015;27(15):2515-2520.
[DOI] -
14. Nguyen TB, Nakanotani H, Hatakeyama T, Adachi C. The role of reverse intersystem crossing using a TADF-type acceptor molecule on the device stability of exciplex-based organic light-emitting diodes. Adv Mater. 2020;32(9):1906614.
[DOI] -
15. Yang J, Guo QX, Ren Z, Chen J, Peng Q, et al. Rational molecular design for efficient exciton harvesting, and deep-blue OLED application. Adv Opt Mater. 2018;6(15):1800342.
[DOI] -
16. Li W, Li B, Cai X, Gan L, Xu Z, Li W, et al. Tri-spiral donor for high efficiency and versatile blue thermally activated delayed fluorescence materials. Angew Chem Int Ed. 2019;58(33):11301-11305.
[DOI] -
17. Pei R, Xu Y, Miao J, Peng H, Chen Z, Zhou C, et al. A tetrahedral bisacridine donor enables fast radiative decay in thermally activated delayed fluorescence emitter. Angew Chem Int Ed. 2023;62(13):e202217080.
[DOI] -
18. Jin J, Duan C, Jiang H, Tao P, Xu H, Wong WY. Integrating Asymmetric O-B-N unit in multi-resonance thermally activated delayed fluorescence emitters towards high-performance deep-blue organic light-emitting diodes. Angew Chem Int Ed. 2023;62(18):e202218947.
[DOI] -
19. Frisch MJ, Trucks GW, Schlegel HB, Scuseria GE, Robb MA, Cheeseman JR, et al. Gaussian 16, Revision C.01 [software]. Gaussian, Inc. 2016.
-
20. Xu J, Wu X, Li J, Zhao Z, Tang BZ. Regulating photophysical property of aggregation-induced delayed fluorescence luminogens via heavy atom effect to achieve efficient organic light-emitting diodes. Adv Opt Mater. 2022;10(7):2102568.
[DOI] -
21. Huang R, Chen H, Liu H, Zhuang Z, Wang J, Yu M, et al. Creating efficient delayed fluorescence luminogens with acridine-based spiro donors to improve horizontal dipole orientation for high-performance OLEDs. Chem Eng J. 2022;435:134934.
[DOI] -
22. Fu Y, Liu H, Yang D, Ma D, Zhao Z, Tang BZ. Boosting external quantum efficiency to 38.6% of sky-blue delayed fluorescence molecules by optimizing horizontal dipole orientation. Sci Adv. 2021;7(43):eabj2504.
[DOI] -
23. Zhu T, Yang Y, Zheng L, Becker ML, Gong X. Solution-processed flexible broadband photodetectors with solution-processed transparent polymeric electrode. Adv Funct Mater. 2020;30(15):1909487.
[DOI] -
24. Lin G, Peng H, Chen L, Nie H, Luo W, Li Y, et al. Improving electron mobility of tetraphenylethene-based AIEgens to fabricate nondoped organic light-emitting diodes with remarkably high luminance and efficiency. ACS Appl Mater Interfaces. 2016;8(26):16799-16808.
[DOI] -
25. Park SR, Kim SM, Choi Y, Lee JY, Lee JH, Suh MC. The influence of dipyridylamine-carbazole based bipolar host materials for green PHOLEDs. Dyes Pigm. 2019;170:107621.
[DOI] -
26. Hu B, Cai X, Li C, Huang W, Ichikawa M. New xanthone derivatives as host materials: improvement of carriers balance for high-efficiency green phosphorescent OLEDs using two host materials. Dyes Pigm. 2020;178:108333.
[DOI] -
27. Yu M, Wu X, Liu H, Yang Z, Qiu N, Yang D, et al. Improving electroluminescence efficiency by linear polar host capable of promoting horizontal dipole orientation for dopant. Adv Sci. 2023;10(6):2206420.
[DOI]
Copyright
© The Author(s) 2025. This is an Open Access article licensed under a Creative Commons Attribution 4.0 International License (https://creativecommons.org/licenses/by/4.0/), which permits unrestricted use, sharing, adaptation, distribution and reproduction in any medium or format, for any purpose, even commercially, as long as you give appropriate credit to the original author(s) and the source, provide a link to the Creative Commons license, and indicate if changes were made.
Share And Cite