- College of Biomedical Engineering, Sichuan University, Sichuan 610065, Chengdu, China.
Abstract
Brain diseases, including psychosis, neurological disorders, strokes, etc., account for more than 15% of all global health damage, which is higher than that caused by cancer and cardiovascular diseases. Brain health and the treatment of brain diseases have become major challenges of the 21st century. The past few decades have witnessed a series of significant advances in human brain science research. The pathogenesis of brain diseases at the molecular and genetic level is being revealed, indicating promising outcomes. However, the existence of the blood-brain barrier (BBB) significantly impedes the delivery of drugs and genes to the brain, which seriously hinders the treatment of brain diseases. This review article provides a brief overview of the concept and history of the BBB. We focus on the critical obstacles and solutions of different kinds of therapeutics, including small molecule drugs, peptides, proteins, and genes, to break through the BBB. Delivery mechanisms, strategies, and vehicles are summarized. Recent advances and efforts in drug delivery studies that aim to overcome the BBB will greatly facilitate the development of brain disease treatment.
Keywords
1. Introduction
According to the World Health Organization (WHO), brain diseases account for an increasing proportion of all human diseases. It is predicted that brain diseases are becoming a major challenge in the next few decades[1]. One of the challenges in treating brain diseases is that many valid therapeutics in other tissues fail because of their inability to effectively cross the blood-brain barrier (BBB). The BBB refers to the barrier between plasma and brain cells formed by brain capillary wall and glial cells, and the barrier between plasma and cerebrospinal fluid formed by the choroid plexus[2]. The unique mechanisms of cellular exchange between the blood, brain, and cerebrospinal fluid protect the brain from harmful substances, but they also make brain-targeted drug delivery difficult[1]. Although many advanced drug delivery strategies have been developed, the efficiency and accuracy of drugs crossing the BBB still remain unsolved. To meet the challenges related to the delivery of drugs to the central nervous system (CNS), scientists are devoting much time and effort to fully understanding the mechanisms of the BBB. Studies have revealed that there are different functional characteristics between normal tissue barriers and the BBB[3], as well as between the BBB under normal physiological conditions and pathological conditions[4-6]. The major factors affecting this mechanism have been identified. However, this is just the first step towards the development of drug delivery system targeting the BBB. To break through the BBB and deliver drugs to the targeted brain tissues, it is often necessary to introduce a well-designed drug delivery system.
In this review, we briefly introduce the discovery of the BBB, dialectically point out its double-edged function, provide a comprehensive understanding of the challenges and solutions for different medications to overcome the BBB, and propose some ideas on the future development of brain-targeted drug delivery systems.
2. The Blood-brain Barrier
2.1 BBB: discovery, structure, and the function
The discovery of the BBB dates back to the end of the 19th century. When Paul Ehrlich intravenously injected aniline dye to rats, it was found that most organs of the rats were stained by aniline, except for their brains. Years later, Paul's student Edwin Goldman injected the dye directly into the spinal fluid of rats. This time, the brain cells were successfully stained; however, other tissues and organs of the rats remained intact. Therefore, it was believed that there existed some kind of compartmentalization between the brain and the rest of the body, which was named the BBB by Dr. Lewandowski. Although the term BBB remains in use today, it was generally believed at that time, due to limited technological conditions, that the barrier between the brain and other tissues was caused by the fine blood vessels around the brain. The knowledge about the BBB became clear over time. Studies in 1955 indicated that the BBB was formed by a glial sheath covering the brain capillaries. However, later research in 1967 revealed that the "barrier" resulted from tight junctions (TJs) between capillary endothelial cells in the CNS[7].
To date, it is well recognized that the BBB is a dynamic interface between blood and brain tissue that selectively blocks the passage of substances. For example, the BBB allows water, oxygen, and small lipophilic components to enter the brain from the blood, but blocks toxins, pathogens, and other potentially harmful substances. Physiologically, the BBB is composed of endothelial cells, pericytes, astrocytes, and junctional complexes including TJs and adhesive junctions (Figure 1). The core component of the BBB structure is the TJs of brain capillary endothelial cells. Different from capillaries in other organs and tissues, capillaries in the brain lack small transcellular pores and instead fuse closely with one another to form TJs and surrounded by a continuous basement membrane, restricting the exchange of substances between blood and brain tissue[3,8]. Pericytes are mural cells arranged at intervals along the capillary wall, embedded in the basement membrane. Astrocytic end feet outside the basement membrane completely surround about 85% of the surface of brain capillaries, forming a multilayer membrane structure of the brain capillaries and constituting a protective barrier of the brain tissue. Pericytes and astrocytes are thought to be associated with signals that promote the TJs necessary for endothelial cells to establish the BBB. The surface area of the BBB in an adult is between 12 and 18 m2[2]. Considering the large surface area, together with the close distance of less than 25 μm between capillaries and brain cells, the BBB becomes an important functional structure for maintaining the normal physiological state of the CNS[2].
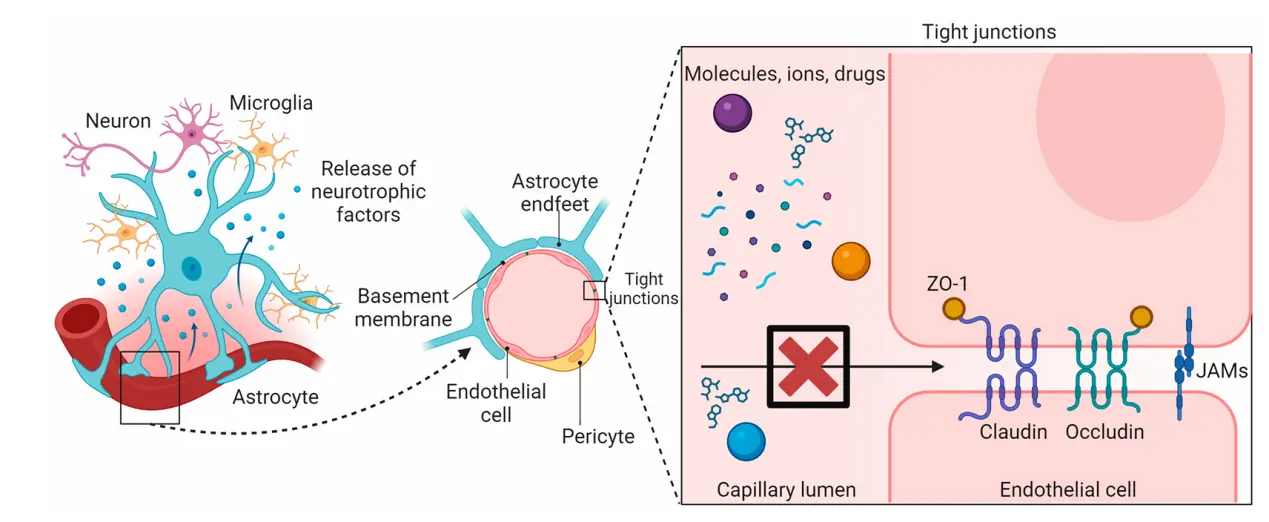
Figure 1. The structure of the BBB. The BBB is composed of endothelial cells, pericytes, astrocytes, and junctional complexes including TJs and adhesive junctions[8]. BBB: blood-brain barrier.
2.2 BBB: hinder or defender?
The BBB controls the transportation of ions and chemicals from the circulation system to the brain tissue in normal physiological circumstances[9], thus providing a controllable microenvironment to maintain homeostasis and a physical limitation on the potential toxins. For example, Na+, K+, Ca2+, and Cl−, as the main ions in the CNS[2,9], play an important role in maintaining the function of nerve and synaptic signal transduction. The levels of these ions in the CNS should be maintained at a constant concentration, which is mainly dependent on the ion transporters of the BBB to control their efflux and influx processes[6]. In fact, this example is just the tip of the iceberg. The poor permeability of the BBB is particularly reflected in that it only allows lipophilic molecules with a molecular weight less than approximately 500 Da to enter the brain through passive diffusion[10,11]. Nutrients, including glucose, amino acids, and fatty acids, are critical for maintaining CNS function. However, they can only enter the brain through specific solute carrier (SLC) transporters[5,9].
For the chemotherapy of brain diseases, including brain cancers and neurodegenerative diseases such as Parkinson's disease (PD), Alzheimer's disease (AD), schizophrenia, etc., the BBB has become an obstacle. The BBB limits the entry of most chemotherapeutics into the CNS. The low bioavailability of brain-targeted drugs not only leads to poor therapeutic effects, but also has severe side effects due to accumulation in other tissues and organs. If the BBB can be seen as a shield, then materials that can penetrate the BBB may be compared to a long-handled weapon, such as a spear. For decades, scientists have devoted themselves tirelessly to seeking the "spear"-the strategies for assessing BBB penetration and facilitating brain drug delivery.
It is worth noting that under pathological conditions, the structure and function of the BBB may be compromised, which could provide opportunities for drug delivery[8]. Evridiki et al. summarized the dysfunctional BBB in pathological conditions including stroke, traumatic brain injury (TBI), and neurodegenerative disorders[8]. The ion dysregulation, edema, and neuroinflammation triggered by BBB disruption lead to neuronal dysfunction, heightened intracranial pressure, and neuronal degeneration, instead enabling nanoparticle-mediated drug delivery (Figure 2).
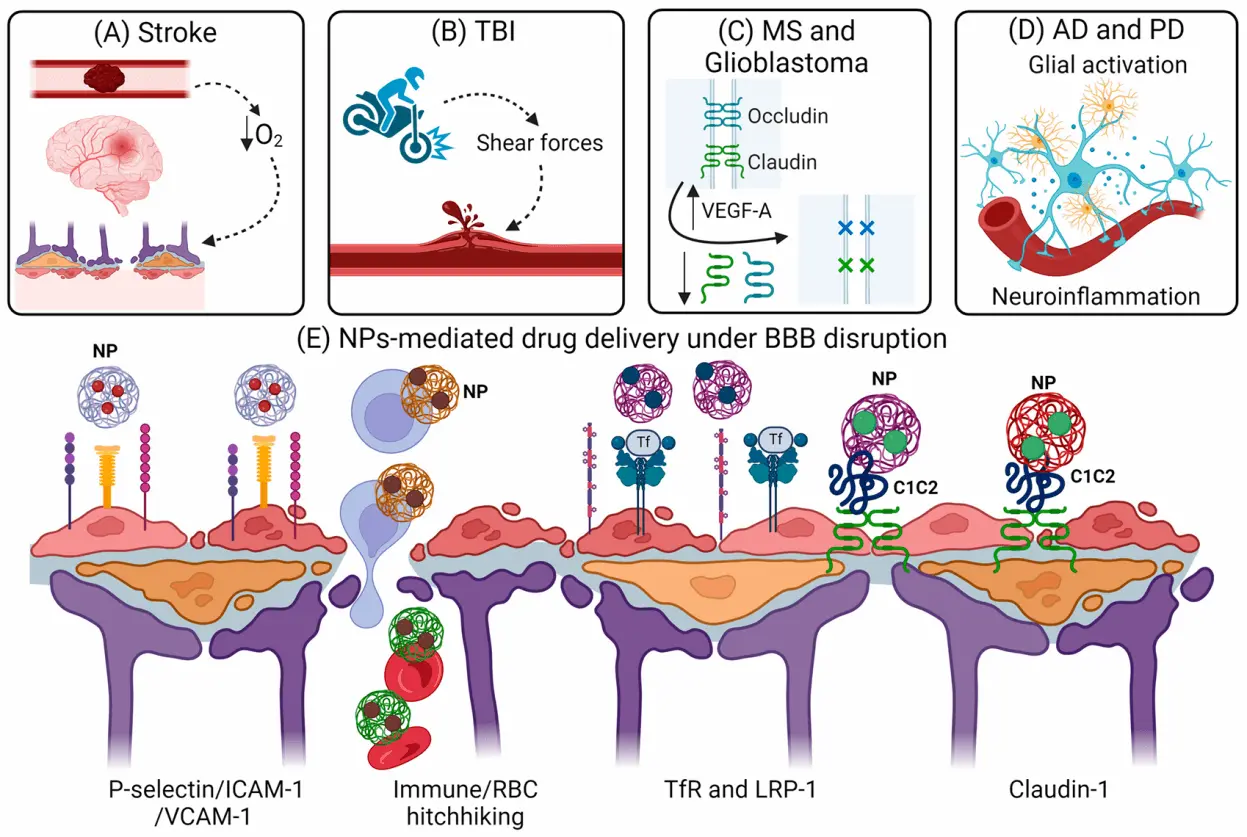
Figure 2. BBB disruption under pathological conditions and NP-mediated drug delivery. (A-D) Mechanisms of BBB disruption in (A) stroke due to increased oxidative stress, (B) TBI due to shear force-induced mechanical injury, (C) MS and glioblastoma due to upregulation of VEGF-A and downregulation of tight junction proteins, and (D) AD and PD due to inflammatory cytokine release and neuroinflammation. (E) Leveraging BBB alterations under pathological conditions to facilitate nanoparticle delivery across the BBB[8]. TBI: traumatic brain injury; MS: multiple sclerosis; AD:Alzheimer's disease; PD: Parkinson's disease; NPs: nanoparticles; BBB: blood-brain barrier; RBC: red blood cells; TfR: transferrin receptors; LRP: low-density lipoprotein receptor-related protein.
3. The Challenges and Solutions for Different Medications to Overcome the BBB
In order to regulate the entry and exit of intracellular metabolites, brain microvascular endothelial cells (BMECs) exhibit specific transport and receptor proteins, as well as an extremely low frequency of vesicle-mediated endocytosis. Additionally, attributed to the numerous transmembrane proteins, such as TJs and adhesive junctions that are expressed on the surface of BMECs, the flow of molecules in the blood around the cells is noticeably restricted, resulting in decreased transcellular and paracellular flux across the BBB[12]. Astrocytes and pericytes also enhance the integrity of the BBB through physical support and the secretion of bioactive substances[13]. Since very few lipophilic small molecular medicines can penetrate the BBB and reach effective brain concentrations, it poses significant challenges for the diagnosis and treatment of CNS diseases.
We reviewed the challenges and current solutions for different types of drugs in crossing the BBB.
3.1 Small molecule drugs
The physiological barrier of the BBB successfully prevents nearly all large molecules from entering the brain, and only 2% of small molecule drugs can penetrate the BBB to exert therapeutic effects[14]. For example, in comparison with trastuzumab, a monoclonal antibody (mAb) with a molecular weight (MW) of 185 kDa, small molecule tyrosine kinase inhibitors (TKIs) such as lapatinib, afatinib, and erlotinib, are superior at crossing the BBB[15], offering several advantages in the treatment of brain metastasis associated with human epidermal growth factor receptor 2 (HER2)-positive tumors[16]. Among the anti-epidermal growth factor receptor (EGFR) drugs approved by the FDA for clinical use, lapatinib (Tyverb®) stands as the only member in this category[17]. In fact, between 2016 and 2020, small molecules accounted for the bulk of novel medicines that the FDA approved for the prevention, detection, or treatment of CNS diseases. Therefore, the development of additional novel small molecule drugs for CNS disorders is of practical importance.
Passive diffusion is the predominant mechanism for transporting small molecules into the brain. The transcellular free diffusion through the phospholipid membranes of BMECs is regulated by many physicochemical properties of lipophilic small molecules, including lipophilicity, MW, hydrogen bonding, polar surface area, and charge[18]. It is more probable for molecules within the MW range of 400 Da to 500 Da to experience substantial free diffusion, which is characterized by an inverse relationship between MW and the diffusion coefficient[19]. The polarity of small molecule drugs is another factor that restricts their ability to cross the BBB. An increase in the number of hydrogen bonds within a drug molecule enhances its hydrophobicity, thereby reducing its interaction with the cell membrane and subsequently reducing its passive diffusion. Therefore, to facilitate transcellular free diffusion across the BBB, molecules need to comply with the MW criterion of less than 400-500 Da and the hydrogen bond criterion of fewer than 7[20]. Nevertheless, a large number of small molecule candidate medications do not possess these attributes, thus impeding the development of treatments for CNS disorders. According to research data, a minority of small drug molecules approved by the FDA, specifically fewer than 2%, have varying degrees of ability to penetrate the entire BBB. Consequently, enhancing the capacity of small molecules to pass through the BBB and advancing the creation of novel medications specifically designed for the brain has considerable practical implications in the realm of treating CNS-associated disorders.
There has been significant progress in the development and investigation of various delivery techniques for small molecule drugs. These strategies can be broadly categorized into four types: (1) enhancement of drug physicochemical properties to facilitate passive diffusion, (2) modification of drug structure for delivery through the carrier-mediated transport (CMT) system, (3) disruption of TJs between cells, and (4) utilization of drug delivery systems such as nanoparticles and viral carriers, which will be discussed in particular in Section 4. It is noteworthy that further caution must be exercised when employing methods such as physical ultrasonography or hypertonic chemicals to disrupt TJs, since these approaches can frequently result in toxicity or impairment of brain function[21].
Modifying the physicochemical properties of drugs is essential to maximize brain exposure levels of small molecule drugs. Enhancing the lipophilicity of medications by inhibiting hydrogen bonding can encourage their penetration into the CNS. Codeine, for example, can be generated by converting the hydrophilic 3-hydroxy group of morphine into a more lipophilic methoxy group. Similarly, the synthesis of heroin involves the acetylation of both the 3- and 6-hydroxyl groups of morphine[22]. Heroin shows a much higher degree of lipophilicity in comparison to codeine, whereas codeine displays a noticeably higher level of lipophilicity than morphine. The heightened lipophilicity gives rise to a notable increase in the absorption of heroin by the rat brain, surpassing the uptake of morphine by over 30 times[23]. Xamoterol is categorized as a β -1 adrenergic receptor agonist. Yi et al. altered the amide functional group of xamoterol to an ether group with lowered polarity, and the resultant substance was named STD-101-D1[24]. In contrast to xamoterol, STD-101-D1 demonstrates enhanced permeability across the CNS. Moreover, it has been observed that the brain concentration of STD-101-D1 exhibits a 22-fold increase compared to xamoterol after intravenous injection. However, the augmentation of lipophilicity has the risk of influencing the efficacy of medicinal properties[25]. Therefore, the strategy of increasing the lipophilicity of small molecule drugs to enhance BBB penetration is extremely limited in practical applications.
There are other ways for small molecule drugs to enter the brain besides passive diffusion. CMT is a phenomenon wherein small molecule compounds, including hexose, amino acids, nucleosides, vitamins, and hormones, move across the BBB by utilizing specialized transporter proteins[26]. This process occurs along the concentration gradient of these substances and does not require the expenditure of energy. Transporter proteins exhibit substrate selectivity and have a capacity of saturable transport. The glucose transporter type 1 (GLUT1), which accounts for over 90% of all glucose transport proteins at the BBB, can function as a conduit for small molecule drugs to penetrate the brain[27]. The medications with the help of GLUT1 transporter can be categorized into two groups: glucose drug conjugates and glucose mimic pharmaceuticals[28]. Medicines or peptides with limited BBB penetration can be conjugated with a hydroxyl group on D-glucose. Zhao et al. conjugated venlafaxine with D-glucose and formed a new reagent, V-TDS-G[29]. Compared with venlafaxine, V-TDS-G exhibited better targeting ability and considerably boosted the level of venlafaxine in the brain. The relative uptake efficiency and concentration efficiency reached 5.69 and 5.70 times that of the unmodified form of venlafaxine, respectively. Glucose-mimic pharmaceuticals maintain the fundamental structure of D-glucose molecules while incorporating certain substituents to provide drug-like characteristics or augment their affinity for GLUT1, thus promoting their bioavailability. Furthermore, it has been suggested that L-type amino acid transporter 1 (LAT1) and the equilibrative nucleoside transporter 1 (ENT1) may serve as conduits for the entry of circulating small molecule medicines into the brain[30,31].
3.2 Peptides and proteins
The BBB presents a substantial obstacle to the effective administration of macromolecular drugs, such as peptides, recombinant proteins, and therapeutic antibodies, which are widely utilized in the biomedicine domain[32]. Peptide and protein medicines are often administered through two routes, namely oral ingestion and intravenous injection. The oral delivery of drugs is affected by the presence of trace digestive enzymes in the oral cavity. Upon passing through the esophagus and entering the gastrointestinal system, medication absorption may be drastically impeded by alterations in pH levels and the integrity of the gastrointestinal epithelial mucosa. Protein-based pharmaceuticals administered orally must traverse the digestive system and epithelial cell barriers before reaching the bloodstream, whereas intravenous delivery allows for immediate entry into the bloodstream[33]. In the context of CNS diseases, pharmaceutical agents that are introduced into the circulatory system encounter difficulties related to immune identification and renal clearance. Additionally, these agents suffer impediments posed by the BBB, a biological barrier that presents tremendous challenges to the prompt therapy of brain disorders. For illustration, monoclonal antibodies that have been engineered to target cytotoxic T lymphocyte antigen-4 (CTLA-4) and programmed cell death protein-1 (PD-1), which regulate the immune system response to combat and manage the malignant growth of cancer cells, have received FDA approval[34]. However, their effectiveness in treating brain tumors is diminished as a result of their inability to permeate the BBB. Evidence has shown that the presence of loss-of-function variants of TREM2 is linked to a heightened susceptibility to Alzheimer's disease (AD)[35]. Activation of innate immune receptor may be a valuable treatment. Still, the effectiveness of exploiting TREM2-activated antibodies is hindered by the confined transport across the BBB[36]. The primary etiology of PD is postulated to be the degeneration of dopaminergic neurons within the densely populated substantia nigra, causing a reduction of dopamine concentrations within the striatal region[37]. Certain investigations are focused on the endeavor of regenerating dopaminergic neurons[38,39]. Protein-based therapies have demonstrated promising potential in various therapeutic applications, particularly in the context of neurotrophic factors. Notably, glial cell-derived neurotrophic factors (GDNF), brain-derived neurotrophic factors, insulin-like growth factors, and other related factors have exhibited vital therapeutic prospects. Nevertheless, these compounds are likewise afflicted by a notable deficiency in BBB permeability. Hence, research on the transportation of peptides and proteins to the brain holds considerable promise for expediting advancements in the treatment of brain diseases. Galstyan et al. described the targeted nanoscale immunoconjugates on natural biopolymer scaffold, with covalently attached a-CTLA-4 or a-PD-1 for systemic delivery across the BBB and activation of local brain anti-tumor immune response (Figure 3)[34].
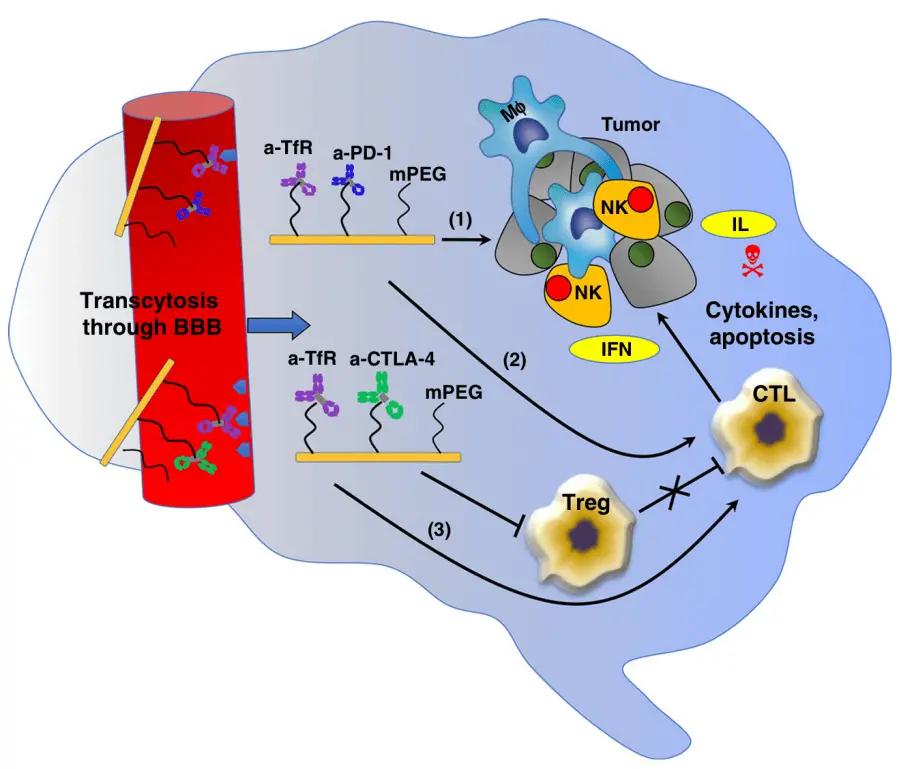
Figure 3. The proposed mechanism of synergistic treatment with a-CTLA-4 and a PD-1 mAbs when they cross the BBB as part of a nano immunodrug[34]. TfR: transferrin receptors; PD-1: programmed cell death protein-1; mPEG: monomethoxy poly(ethylene glycol); NK: natural killer; IFN: interferon; IL: interleukin; Treg: Regulatory T cell; CTL: cytotoxic T lymphocyte; BBB: blood-brain barrier.
Actually, receptor-mediated endocytosis (RMT) fosters the entry of some endogenous macromolecular peptides and proteins from plasma into the brain. This presents an intriguing avenue for macromolecular medications to overcome the BBB and access the CNS[40]. Transferrin receptors (TfR), particularly TfR1, are present on the surface of BMECs and are extensively utilized as a prominent target[41]. At physiological pH, iron displays a strong affinity for transferrin (Tf), triggering the formation of holo-Tf. This complex is then internalized into BMECs via TfR-mediated endocytosis[42]. In the acidic environment, iron is liberated, enabling the transformation of iron-bound Tf into its apo form (Apo-Tf). The Apo-Tf molecule forms a complex with the TfR since it needs to be re-inserted into the plasma membrane. Once the extracellular environment becomes neutral, the Apo-Tf dissociates from the TfR and a new cycle is initiated[43]. It has been demonstrated that Tf is capable of crossing the BBB[44]. However, the use of endogenous Tf as a carrier is not feasible due to its high concentration in serum and the near-complete saturation of TfR with Tf. Hence, specific peptides or MAbs that exhibit affinity towards epitopes located on receptors of the BBB have emerged as a more favorable option. The proposal of the molecular trojan horse (MTH) concept was based on the aforementioned information. Trojan horse vehicles, which are conjugated with macromolecules, like peptides or proteins, facilitate the transportation of cargo into the brain by selectively attaching to a specific spot on the receptor[45]. Of note, the spatial characteristics of this site are supposed to differ from those of the binding site for endogenous ligands. Erythropoietin (EPO) is a hematopoietic growth factor weighing around 30.4 kDa. It exhibits neuroprotective and regenerative properties, making it a potential therapeutic approach for improving the condition of AD[46]. Zhou et al. developed a fusion protein known as MTH-EPO[47]. This fusion protein was created by utilizing a rat/mouse antibody that specifically targeted the mouse TfR (cTfRMAb) and EPO receptors. The interaction between the fusion protein and the TfR improves the delivery of EPO to the brain by crossing the BBB. Simultaneously, the connection of fusion protein with the EPO receptor in the brain leads to the manifestation of neuroprotective effects induced by EPO. Moreover, the cTfRMAb-EPO exhibits an increased elimination ratio from the systemic circulation as compared to EPO. It is anticipated that this mechanism will mitigate the hematological deleterious effects commonly associated with elevated levels of circulating EPO. At present, there have been debuts of products that are based on TfR. Pabinafusp alpha (JR-141) refers to a fusion protein consisting of the TfR antibody and lysosomal enzymes, used in the management of mucopolysaccharide storage syndrome type II (MPS II). This therapeutic intervention represents a milestone as it is the first enzyme replacement medication to receive regulatory approval, which have access to permeate the BBB. In conjunction with ligand modification, Kariolis et al. employed a methodology known as antibody transport vehicle (ATV) to manipulate and alter the antibody Fc, which permits the transportation of antibody across the BBB[48]. In essence, the methodology involves employing stochastic genetic alterations to generate a diverse repertoire of variants within a yeast display library. Subsequently, the library is subjected to screening using the human transferrin receptor (hTfR) as a target, with the objective of identifying molecules capable of binding to hTfR and facilitating cellular entry, which is conducted by flow cytometry. In the context of in vivo investigations conducted on mice, it has been confirmed that the modified BACE1 antibody demonstrated a notable ability to overcome the BBB and gained access to the brain. Following a single dosage of 50 mg/kg over a 24-hour period, the brain exposure of ATV: BACE1 was found to be approximately 40 times that of the anti-BACE1. Furthermore, insulin receptors (InsR) are also a subject of momentous concern. The activation of the tyrosine kinase catalytic domain in cells can be enhanced by InsR through their binding and internalization with insulin, leading to the accomplishment of many physiological functions. The application of insulin as a ligand-coupled pharmaceutical agent can be susceptible to fluctuations in blood glucose levels. Consequently, non-competitive antibodies that selectively bind to InsR appear to be a more viable option. The sole pharmaceutical agent sanctioned by the FDA for the purpose of enzyme replacement therapy in the treatment of MPS II is recombinant idursulfase (recombinant human IDS). Gusarova et al. developed a unique chimeric protein called HIR-Fab-IDS, which was constructed by combining an anti-human insulin receptor Fab fragment with recombinant idursulfase[49]. HIR-Fab-IDS has demonstrated a notable in vitro affinity for insulin receptors in both human and monkey tissues. Furthermore, studies have indicated that radiolabeled products were capable of effectively permeating all regions of the brain as well as peripheral tissues following intravenous injection in monkeys. The successful development of HIR-Fab-IDS not only provides a new strategy for treating specific central nervous system diseases, but also offers an important reference for the design and delivery of other macromolecular drugs, especially in cases where crossing the BBB is required. Similar to the two receptors mentioned earlier, peptides or protein therapeutics can target the brain through receptors such as insulin-like growth factor receptors (IGFRs), leptin receptors (LEPRs), and other targets. However, it is imperative to acknowledge certain concerns pertaining to antibody-targeted therapy. Further research is needed to determine the potential applicability of active targeting therapy in cases where there is significant BBB damage caused by the disease, or to regulate the expression of different receptors in the disease environment.
Indeed, numerous intriguing strategies have emerged for delivering peptide and protein medicines to the brain. Cationic proteins tend to increase cellular internalization via charge-based or adsorption-based endocytosis mechanisms, commonly referred to as adsorptive-mediated transport (AMT)[50]. Hexanediamine was reacted with bovine serum albumin (BSA) at pH values of 6.8 and 7.8, leading to the formation of a highly cationic BSA (cBSA) with an isoelectric point of more than 10, as well as a mild cBSA variant with a pI range of 8.5-9, respectively[51]. The capacity of isolated microvessels derived from bovine brains to bind and internalize moderate amounts of cBSA has been observed. The binding and endocytosis of endorphins by cerebral microvessels, when coupled with cBSA, exhibit vastly improved efficiency. The spatial localization of this peptide within the brain was also evaluated by in vivo experiments. Given this assumption, certain individuals have attempted to introduce a positive charge to the antibody with the aim of augmenting its ability to cross the BBB. The therapeutic function of the AMY33 antibody in the treatment of AD is achieved through its binding affinity to Aβ amyloid peptide within the brain. Bickel et al. found that the dissociation constants of cationic AMY33 and natural AMY33 were determined to be 4.2 ± 0.7 nM and 1.4 ± 0.3 nM, respectively, which suggested that cationic proteins may have the potential to effectively overcome the BBB[52]. However, the extensive implications of this approach on cellular and tissue toxicity, as well as the effects of cationic treatment on the duration of activity and immunogenicity of the antagonist, present limitations on its possible scope of utilization.
3.3 Nucleic acid
Gene therapy, which can be accomplished by introducing therapeutic genes or by replacing, silencing, or correcting faulty genes, has emerged as a novel avenue for the treatment of brain diseases nowadays[53]. A prevalent approach for boosting gene expression is plasmid DNA (pDNA), which can be specially engineered to increase the expression of particular genes. In various PD animal models, GDNF has proven neuroprotective potential. Yurek et al. compressed a single molecule of polyethylene glycol-substituted lysine polymer peptide and plasmid DNA encoding human GDNF into DNA nanoparticles to circumvent the BBB[54]. The results are encouraging because GDNF expression was boosted in the unilateral 6-OHDA rat model by both pDNA-loaded nanoparticles and naked pDNA. Stronger neuroprotective results were observed in the nanoparticles group, although this could be attributed to the easy cyclic disintegration and low absorption of naked pDNA. To encourage uptake by BMECs and prevent nucleic acids from enzymatic destruction, carriers are often necessary for the delivery of therapeutic nucleic acids. After cellular internalization, pDNA needs to pass through the nuclear membrane with the goal of altering the expression of related genes. Molecules with MWs between 40 and 70 kDa can passively diffuse through the nuclear pores, and nuclear localization signals (NLS) can upregulate the efficiency of pDNA transfection. After transfection, pDNA needs to be transcribed into messenger RNA (mRNA), which is then responsible for carrying out the protein's specific function in the cytoplasm. Compared to pDNA, mRNA-based gene therapeutics have a faster development cycle, a lower risk of genomic integration, higher transfection efficiency, and the ability to directly and quickly translate into proteins. Research on mRNA gene therapeutics has opened up new opportunities for crossing the BBB and enhancing the expression of target genes, despite the fact that there are currently only a small number of products in clinical use.
Some nucleic acid-based pharmaceuticals are required to silence the target genes that are overexpressed in CNS disorders. Based on the complementary base pairing principle, antisense oligonucleotides (ASOs) are a class of single-stranded nucleotides that can recognize and bind to specific target RNA sequence. They either degrade the target gene by the action of degrading enzymes like ribonuclease (RNase) or prevent the production of pathogenic proteins by steric hindrance. In the absence of transcription and translation, this process of guiding the expression of related genes is undoubtedly faster and more effective. MicroRNAs (miRNAs) are naturally occurring single-stranded non-coding nucleotides that prevent the expression of specific genes by forming RNA-induced silencing complexes (RISCs) with the target RNA. One strand of the double-stranded RNA known as small interfering RNA (siRNA) binds to mRNA to generate RISCs, which mediate mRNA degradation. Once entering the body, nucleic acids must avoid being cleared by the immune system and hydrolyzed by nucleases in the intracellular milieu. To pass through the BBB, they can be modified to be more hydrophilic, negatively charged, resistant to lysosomal degradation, and safe to prevent the production of harmful genes. The solution to address these needs is designing gene vectors. A spherical nucleic acid (SNA) medication (NU-0129) has been created that targets the glioblastoma (GBM) cancer-causing gene Bcl2L12 with core Au nanoparticles (NPs) covalently coupled to siRNA[30]. With the aid of nanotechnology, this medication is able to selectively target Bcl2L12 and downregulate its expression across the BBB through scavenger receptor (SR)-mediated transcytosis. The veracity of this claim has been corroborated by a phase 0 clinical study[55]. In clinical trials, NU-0129 has been shown to effectively cross the BBB and accumulate in tumor cells, reducing the expression of the Bcl2L12 protein, and thus helping to inhibit tumor growth. Furthermore, encouraged by the clinical trial results of NU-0129, researchers are exploring the use of this technology for the therapy of other neurological diseases.
4. Delivery Vehicles for Brain Drug Delivery
Nowadays, there has been a surge in research interest in implementing drug carriers for delivering drugs specifically to the brain. Brain transfer technology encompasses both invasive and non-invasive methodologies[56]. The latter includes a series of biological methods, focused ultrasound (FUS)[57], nanocarrier systems[58-61], and intranasal delivery[62]. These technologies offer numerous benefits, including non-invasiveness, a high degree of targeting efficiency, and precise control over drug loading and release at the BBB[63]. However, it is important to recognize that some constraints cannot be overlooked in the context of therapeutic applications.
This section provides a framework of several carrier systems, such as polymer nanoparticles, liposomes, dendrimers, exosomes, and viral vectors.
4.1 Strategies to overcome the BBB
There exist two primary mechanisms for traversing the BBB: the paracellular pathway and the transcellular pathway (Figure 4)[64]. Pharmaceutical substances are able to get across the BBB by exploiting diverse transcellular pathways, primarily by leveraging the physiological mechanisms of endogenous molecular transport, which include passive diffusion, CMT, RMT, and AMT. The investigation of these routes has been extensive due to their potential to safeguard the integrity of the BBB. Additionally, the modulation of TJs primarily facilitates the augmentation of drug absorption via the paracellular route.
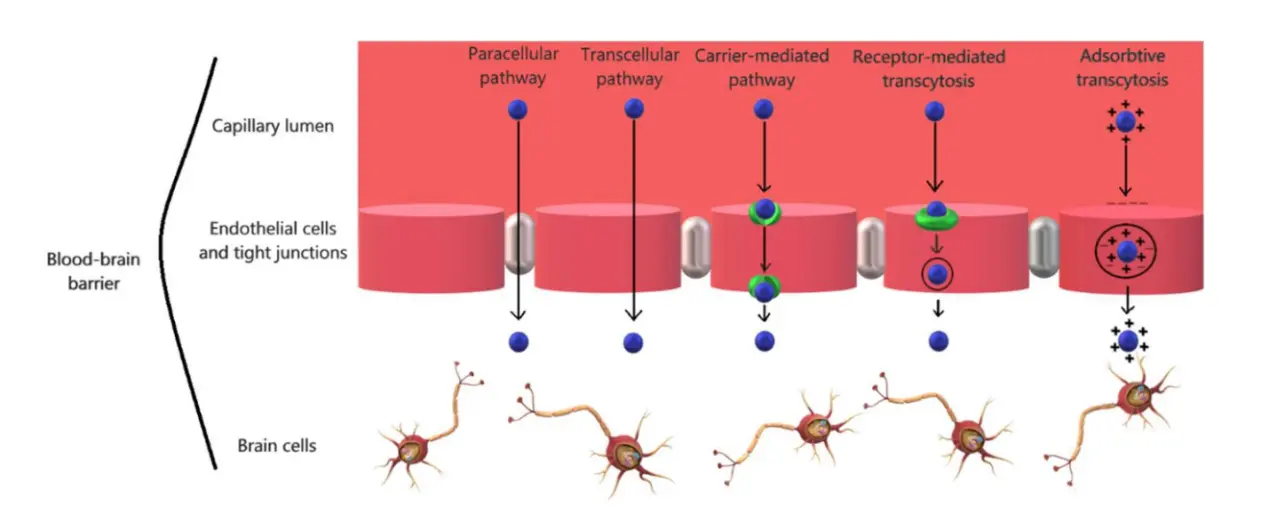
Figure 4. Multiple delivery pathways across the BBB by using nano-carrier[64]. BBB: blood-brain barrier.
4.1.1 Transcellular pathway
Lipophilic small molecules cross the BBB mainly through passive diffusion. Nanocarriers are often applied for the targeted delivery of therapeutic agents to brain tumor cells, mostly emphasizing the enhanced penetration and retention effect (EPR). CMT is also classified as a form of passive transport that operates without consumption of energy. The surface of BMECs harbors a variety of solute carriers (SLCs), such as GLUT1, LAT1, ENT1, choline transporters, monocarboxylate transporters (MCTs), glutathione transporters, and others, which have emerged as potential targets for therapeutic intervention in the treatment of different CNS disorders (as discussed in Section 3.1)[64].
RMT transports medications via the binding of receptor ligands, enabling active brain targeting. This transport method is not only used for protein and antibody therapeutics across the BBB, as explained in Section 3.2, but also serves as a targeted modification scheme for nanocarriers[65]. Zhang et al. developed micelles composed of self-assembled ferrocene (Fc) conjugated D-α-tocopherol polyethylene glycol succinate (TPGS) polymer and amphiphilic copolymer poloxamer. The micelles specifically delivered drugs to the brain. Due to its specific design, the TPGS-Fc carrier may encounter obstruction by efflux pumps, yet it simultaneously preserves a significant level of permeability across the BBB through RMT[66]. Martins et al. set up a multi-ligand PLGA/PEG nanoplatform to deliver docetaxel (DTX) for the treatment of GBM[67]. The pH-responsive Angiopep-2 peptide, serving as the initial ligand, targets the low-density lipoprotein receptor (LDLR) located on the surface of the BBB. The L-histidine (L-His) moiety, serving as the second specific ligand, exhibits binding affinity towards LAT1, which is overexpressed in both the BBB and high-grade gliomas. The combined impact of two ligands dramatically boosts the uptake of medications. When tested in an extracorporeal BBB model, multi-ligand NPs (MLNPs) demonstrated a 3-fold increase in brain transport efficiency compared to normal conditions. In vivo investigations of MLNPs in mice models have shown significantly greater brain accumulation compared to control NPs. Consequently, the discoveries have directly led to a rise of over 50% in the long-term survival rate of mice[67]. These MLNPs enhance their accumulation and targeting in the brain by binding to a variety of different ligands. These ligands can be molecules that target specific receptors on the BBB, promoting the transmembrane transport and cellular uptake of NPs through specific binding to these receptors. In addition to targeting, MLNPs can also utilize mechanisms such as EPR effect, avoiding the action of efflux pumps, utilizing multiple transport pathways, reducing non-specific uptake, and exploiting pathological conditions to work synergistically and improve the accumulation and efficacy of drugs in the brain.
Another drug delivery approach to bypass the BBB is AMT. Transcellular vesicles are produced when anionic domains on the plasma membrane of BMECs electrostatically interact with positively charged molecules. Subsequently, these vesicles fuse with the cellular membrane, thereby permitting the release of chemical substances into the cerebral region[68]. The implementation of the AMT delivery approach relies on the crucial foundation provided by the cationic feature and cell penetrating peptide (CPP) modifications of the carrier surface. The intracellular delivery of proteins, nucleic acids, and other chemicals can be achieved through the application of CPPs. CPPs, composed of less than 30 amino acids, have attracted much attention as they maintain the cell integrity of BBB. CPPs are highly productive instruments for the transportation of pharmacological macromolecules[69]. These peptides usually possess a positive charge and efficiently enter a wide range of cells. The potential interaction between the negatively charged lipids and polysaccharides present on the cell membrane and the positively charged basic amino acids within the CPP is believed to contribute to the passage of the BBB via AMT. Typically, the translocation of small molecules across the lipid bilayer occurs upon their interaction with CPPs. Conversely, when a macromolecule associates with the CPP, transmembrane transport is achieved by the process of endocytosis[70]. Nevertheless, the specific method of internalization remains uncertain. Pang et al. developed a self-assembling nanocomposite consisting of virus-like particles (VLPs) and RNA interference (RNAi) molecules, which featured fluorescence properties. Afterwards, CPP was incorporated to further enhance the BBB penetration. This modified nanocomposite hindered DNA repair processes across the BBB and synergistically interacted with temozolomide (TMZ) to enhance the efficacy of clinical chemotherapy. The integration of these elements has yielded favorable therapeutic outcomes in the treatment of malignant brain tumors[71]. Through positive and negative electrostatic interactions, the affinity between the cargo and BMECs has a binding potential 1000 times greater than that of RMT. It suggests that AMT still holds promise for the advancement of brain drug delivery. On the other hand, it is crucial to note that AMT lacks optimal tissue selectivity due to its tendency for non-specific binding. Therefore, AMT is often employed as a strategy to help break through BBB defenses. Enhancing the tissue selectivity of AMT in brain drug delivery, for example, by leveraging the pathological characteristics of brain diseases to tailor NPs that target brain lesions, can reduce damage to the BBB and further enhance the specificity of brain-targeting NPs, making them more promising in the treatment of brain diseases.
4.1.2 Paracellular pathway
The paracellular pathway is governed by the resistance-enhanced TJs between BMECs, which simultaneously prevents the unrestricted transport of lipids and proteins across the cellular membrane. This mechanism prevents detrimental molecules from entering the brain and also hinders the transport of many pharmaceutical compounds to the brain.
The transient opening of the BBB can be induced by some chemicals such as mannitol and alkyl glycerin. It is imperative to bear in mind that these chemicals exclusively stimulate the opening of the BBB when present at a specific concentration[72]. The barrier will restore its full function once the blood concentration of these substances decreases below the established threshold. The modulation of TJs is additionally regulated by certain receptors. It is widely believed that there are potential therapeutic targets associated with adenosine receptors. Based on empirical evidence, the activation of A1 and A2A adenosine receptors has been observed to improve the BBB permeability in vivo. In order to enhance the specific delivery of various pharmaceutical models to the brain, Gao et al. employed the coupling of the A2A receptor agonist with dendrimers, resulting in the formation of nano-agonists (NAs)[73]. The intravenous administration of NAs strongly strengthened the brain uptake of the macromolecular model drug (with a MW of 45 kDa), as evidenced by in vivo imaging studies. The temporal window of BBB opening can be correlated with the pharmacokinetics of therapeutic drugs through the selection of an appropriate NA, which has an opportunity to optimize drug delivery to the brain.
In addition to employing chemical approaches, several physical stimuli, such as focused ultrasound and microwave radiation, have been found to promptly activate the BBB. The injected microbubbles (MBs) undergo oscillation when subjected to ultrasound, producing mechanical force within the blood vessels and then leading to the opening of the targeted TJs. He et al. developed a bionic drug delivery microbubble named FeDOX@cellMBs[74]. This microbubble was coated with the cell membrane of BMECs and contained a combination of superparamagnetic iron oxide and adriamycin. When deployed in combination with focused ultrasound, it showed proficient glioblastoma localization and absorption. When it comes to clinical treatment, it is important to be cautious about the methodology employed to open the TJs, as it may allow hazardous chemicals into the cerebral region or exacerbate the CNS disorders.
As previously mentioned in Section 2.2, under pathological conditions, small molecules and soluble substances can traverse the BBB through the paracellular pathway, providing possibilities for drug delivery. Additionally, entering the BBB through the paracellular route requires attention to the temporal window of BBB opening. The opening of the BBB is a transient process that may need to be coordinated with the pharmacokinetics of therapeutic drugs to ensure that the drugs effectively reach the brain within the temporal window of BBB opening. On the other hand, to achieve effective drug delivery, precise control of the intensity, timing, and targeted area of physical stimulation is needed to avoid unnecessary side effects.
4.2 Delivery vehicles
The ability of drug delivery vehicles to cross the BBB is primarily related to their size, surface chemistry, biocompatibility, and stability. For example, positively charged nanoparticles can cross the BBB through AMT. Conjugating carriers with targeting ligands on their surface, such as antibodies or peptides specific to certain receptors, can promote RMT or CMT, thereby enhancing their BBB penetration. The biocompatibility of carriers affects their interaction with BBB endothelial cells, which in turn affects their penetration ability. The stability of carriers, including their resistance to enzymatic and chemical degradation, is also critical for successful BBB crossing. In addition, certain specific delivery systems, such as nanoparticles, liposomes, and exosomes, show high BBB penetration efficiency due to their unique physicochemical properties. Delivery carriers can cross the BBB through various mechanisms, including RMT, physical penetration, and by altering the paracellular pathway to increase the permeability. Table 1 summarizes the advantages and disadvantages of different delivery vehicles for crossing the BBB.
Vehicles | Advantages | Disadvantages |
Polymeric nanoparticle | High stability, low immunogenicity, surface modifiability, high capacity for hydrophobic medicines, easy preparation, low cost | Clearance by RES and MPS, imbalance between stability and degradation, unknown in vivo distribution |
Dendrimer | Nanoscale structure, easy modification, membrane affinity | Cytotoxicity |
Liposome | Ability to load both hydrophilic andlipophilic molecules, favorable biocompatibility | Dynamic recombination, difficulty inpredicting potential toxicity in vivo |
Exosome | Low immunogenicity, extended bloodcirculation, efficient BBB penetration,ability to load both, hydrophilic andlipophilic molecules | Low efficiency and high cost of separationand purification, difficulty in achieving highload, unknown connection between extracellular vesicles and the BBB |
Viral vector | Enhanced gene delivery, improved delivery efficiency, reduced cellulardamage | Immunogenicity, insertion mutations, possibility of death, high cost |
RES: Restriction Endonuclease; MPS: Mucopolysaccharidoses; BBB: blood-brain barrier.
4.2.1 Polymeric nanoparticles
Polymer NPs, such as those composed of poly(lactic- co -glycolic acid) (PLGA) and polylactic acid (PLA) NPs, are commonly employed as drug carriers due to their ease of preparation and high capacity for hydrophobic drugs, which extends the duration of medication activity by increasing their half-life and inhibiting their enzymatic degradation within the body[75]. The size of NPs is a crucial factor that determines their ability to get into the brain[76]. The block copolymer, polyethylene glycol- block -polylactic acid (PEG- b -PLA), was synthesized by ring opening polymerization employing PEG as the chain initiator[77]. Then a library of NPs without targeting ligands was developed, encompassing a range of diverse physical and chemical characteristics. The study involved the monitoring of neuronal cell uptake, as well as the assessment of toxicity, endocytosis, and transport in BMECs. Although the manipulation of surface PEG chain length and NP size has no substantial impact on the translocation of the cell barrier, smaller NPs show higher absorption efficiency than larger ones. Furthermore, NPs of comparable dimensions can be employed to illustrate the impact of PEG chain length, whereby NPs possessing longer PEG chains exhibit elevated endocytosis effects[77].
Modification of polymer NPs can improve the BBB permeability, thereby improving the safety and effectiveness of therapeutic interventions. Hou et al. conducted a study aiming at promoting the delivery of lamotrigine (LTG) to the brain for the purpose of treating epilepsy[78]. Six distinct types of PLGA NPs conjugated with different peptides (T7, d-T7, TGN, CGN, GSH, and TAT) to penetrate the BBB were prepared. The resulting NPs were referred to as peptide-lipids@PL. The BBB permeation and selective targeting of neuronal cells of TAT-lipids@PL were maximized. In vivo studies on d-T7-lipids@PL, however, suggest that it has the highest efficacy in the treatment of epilepsy.
Polymer NPs, such as PLGA NPs, can effectively cross the BBB through micropinocytosis and lysosomal pathways[79]. Li et al. reported a PLGA-based nanocomposite that efficiently co-delivered a thermodynamic agent of 2,2'-azobis[2-(2-imidazolin-2-yl) propane]-dihydrochloride and magnetic nanoparticles[80]. This PLGA nanoparticle, by integrating the photodynamic therapy, served as an effective drug delivery system. It not only enhanced the drug's ability to penetrate the BBB but also enable targeted accumulation in the brain lesion area, reducing side effects.
Despite the widespread use of polymeric NPs in the treatment of CNS disorders due to their favorable characteristics, such as high stability, low immunogenicity, and surface modifiability, certain challenges do arise. The insufficient brain tissue delivery resulting from the clearance processes facilitated by the RES and the MPS is critical. Finding the best balance between the stability and degradation of NPs and ensuring that the material is distributed to the intended area still requires a lot of work[81]. For instance, PLGA NPs are negatively charged and frequently modified with PEG to improve their intracellular ability, allowing them to cross the BBB. Furthermore, it is imperative to explore the potential impact of the acidic byproducts generated by the disintegration of PLGA NPs[82].
4.2.2 Dendrimers
Dendrimers, a class of synthetic macromolecules, feature a distinctive topological structure characterized by a highly branched and three-dimensional dendritic architecture[83]. Dendrimers are characterized by the presence of a central nucleus, a layer composed of branching repeating units, and an outer periphery of functional end groups. The transportation of medications to the brain often involves the use of a meticulously designed nanoscale architecture. The process of combining therapeutics with dendrimers can be achieved through either chemical means, such as covalent bonding, or physical means, such as electrostatic interaction, hydrogen bonding, and hydrophobic interactions. To enhance passage across the BBB, a range of functional groups and specific ligands can be introduced onto the surface of dendrimers, particularly at the terminal ends of their branches, which has been reported to significantly enhance the bioavailability and stability of the medicine[84].
Poly(amidoamine) (PAMAM) is a widely recognized dendrimer that finds extensive application in the field of drug delivery. At the physiological pH range, amino-terminated PAMAM is cationic because of protonation, thus enhancing the interaction with negatively charged cell membranes[85]. Moscariello et al. synthesized a biological composite consisting of the streptavidin adaptor and PAMAM dendrimer generation 3 (G3) (Figure 5)[86]. The transfer of dendronized streptavidin (DSA) from the bloodstream to the brain via AMT is facilitated by the positive charge of the PAMAM shell, the outcome of which is the attainment of a concentration of G3-DSA in brain tissue at a level of 0.44% ± 0.03 μg/g. PAMAM is also a highly promising nucleic acid carrier. Liyanage et al. conducted a conjugate, D-siGFP, by covalently coupling a hydroxyl-terminated PAMAM dendrimer (PAMAM-G6-OH) with siRNA[87]. PAMAM dendrimers have been shown to increase the resistance to nucleases and improve delivery efficiency, enabling D-siGFP to cross the BBB without necessitating supplementary modifications. As a result, in a mouse model of in situ glioblastoma, the D-siGFP conjugate effectively knocked down GFP production, demonstrating the astonishing impact of these innovations.
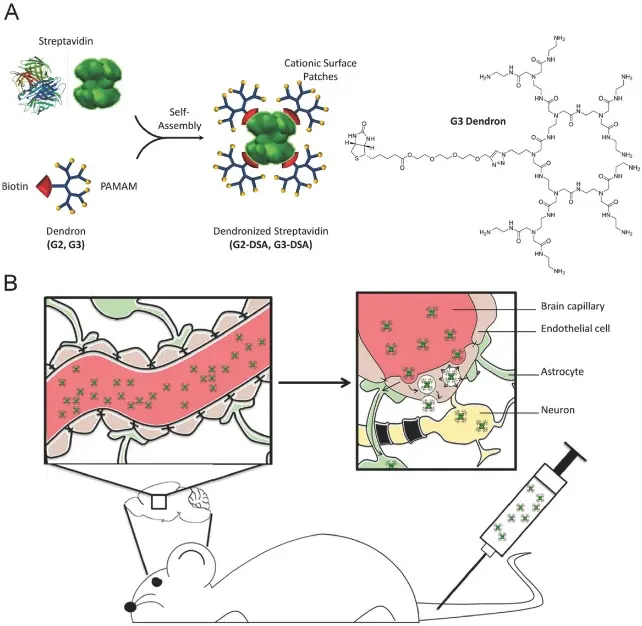
Figure 5. A) Scheme of dendronized streptavidin assembly with detailed G3 dendron structure. B) Schematic illustration of in vivo study design for G3-DSA transport from bloodstream to the brain[86]. PAMAM: Poly(amidoamine). DSA: dendronized streptavidin.
One prominent issue associated with PAMAM is cytotoxicity. The in vivo toxicity of cationic PAMAM dendrimers is mostly influenced by their generation and surface properties[88]. The presence of positive surface charges has been observed to potentially induce cellular demise and diminish cellular viability[89]. The PEGylated PAMAM dendrimers have drastically reduced toxicity due to the shielding of cationic amine terminals by PEG chains. The increase of PAMAM dendrimer generation will elevate its multivalence, but it will concurrently lead to an escalation in toxicity. Therefore, achieving a balance between dendrimer generation and cytotoxicity reduction during cellular interactions is imperative[90].
4.2.3 Liposomes
Liposomes are spherical vesicles consisting of one or more lipid bilayers. Hydrophilic cargo can be loaded in the aqueous compartment of the liposome, and lipophilic molecules can be embedded within their lipid bilayer[91]. Furthermore, they possess the ability to effectively convey bioactive compounds such as enzymes or nucleic acids. Liposome-based drug delivery systems are commonly employed in the diagnosis and treatment of neurological disorders owing to their favorable attributes such as biocompatibility, biodegradability, low toxicity, and the ability to accommodate both hydrophilic and hydrophobic drugs[92].
Taking advantage of AMT, enhancement of brain absorption is promoted by the electrostatic interaction between negatively charged cell membranes and cationic liposomes[93]. Liu et al. constructed a collection of ionizable cationic lipid nanoparticles with diverse amino groups for the purpose of facilitating targeted delivery of siRNA to the brain[94]. The lipid 9-o16b, sometimes referred to as bampa-o16b, was selected as the most potent delivery vehicle due to its high potency. It had a pKa value of approximately 6.5. The accumulation of Cy5-siNc in the brain was higher than 0.1% 24-h post intravenous injection of bampa-o16b/Cy5-siNc. This percentage was notably greater than the levels observed in the lung, heart, and spleen. The results imply that bampa-o16b demonstrates a considerable degree of BBB permeability. The systemic administration of bampa-o16b/siRNA liposomes has demonstrated an efficient delivery of siCD47 and siPDL1, hence presenting substantial opportunities for the advancement of immunotherapy for brain tumors. Besides possible cytotoxicity, the non-specific absorption of peripheral tissues and their binding to serum proteins are also issues for cationic liposomes. Consequently, the utilization of a large number of carriers becomes necessary to achieve the desired therapeutic effects.
In an effort to optimize blood circulation and facilitate targeted delivery to the brain, liposomes can be modified with large molecules such as synthetic polymers, polysaccharides, peptides, antibodies, and similar compounds[95]. Li et al. generated cationic liposomes modified with the targeted peptide angiopep-2, aiming to deliver DOX, YAP-siRNA, and Au NRs to glioma cells for a combined treatment of chemotherapy, gene therapy, and photothermal therapy[96]. The findings of this study are of profound significance for the field of multi-mechanism tumor combination therapy, as well as for the development of BBB delivery methods that bridge the gap between RMT and AMT. Ismail and colleagues devised a liposome nanoplatform functionalized with ApoE[97]. This nanoplatform employs TMZ and artesunate phosphatidylcholine (ARTPC) as components, resulting in the formation of ApoE-ARTPC@TMZ. The ApoE ligands were used to selectively target LDLRs overexpressed in BMECs and GBM cells.
However, it is challenging to predict the in vivo toxicity of liposomes because their size may undergo dynamic recombination, changing their properties over time[98]. Moreover, it is difficult to accurately predict the potential toxicity of liposomes by comparative analysis with previous studies due to the lack of reference materials and well-defined toxicity assays[99].
4.2.4 Exosomes
Extracellular vesicles (EVs), particularly exosomes, have been employed as a novel vehicle for the delivery of genes or drugs[100]. EVs are actively released by cells, with a typical diameter ranging from 30 to 100 nm[101]. They feature an amphiphilic characteristic, rendering them proficient at delivering drugs regardless of their hydrophobic or hydrophilic nature[102]. Additionally, EVs serve as vehicles for transporting a wide range of biological cargo, including miRNA, siRNA, and proteins, to target cells with specificity, benefiting from their low immunogenicity, extended blood circulation half-life, and efficient penetration through biological barriers such as the BBB[103].
The ways in which exosomes interact with the BBB include non-specific extracellular endothelial interaction, RMT, and additional pathways. Unmodified Raw264.7 exosomes are capable of traversing the BBB[104]. In animals with LPS-induced brain inflammation, exosomes flow unidirectionally into the brain at a rate of 3 times that of healthy mice, indicating that inflammation could promote this flow without damaging the BBB. Applying diverse technological advancements to enhance target identification, biological dispersion, and traversal of the BBB would undoubtedly augment the therapeutic capacity of exosomes[105]. The amounts of exosomes absorbed by the brain are doubled when rabies virus glycoprotein (RVG) binds to their surface. As opposed to naked siRNA and untargeted exosomes, the injection of RVG-modified exosomes caused a considerable decrease in GAPDH mRNA levels in numerous brain regions in a study on the delivery of GAPDH siRNA by exosomes. Kim et al. developed exosomes modified with transferrin receptor binding peptide T7 (T7-exo) for the treatment of glioblastoma[106]. These exosomes were designed to carry antisense miRNA oligonucleotides-21 (AMO-21) specifically targeting miR-21. In vitro investigations have demonstrated that T7-exo exhibits superior transport performance on glioblastoma cells compared to unmodified (Unmod-exo) and RVG-modified exosomes (RVG-exo). In vivo delivery studies showed that T7-exo had higher efficacy in delivering AMO-21 to the brain rather than Unmod-exo and RVG-exo[106].
Despite the promising outcomes yielded by current research, some issues must be addressed prior to the application of EVs in the treatment of CNS diseases[107]. There is an urgent need to develop techniques for the isolation and purification of EVs. The features of exosomes are notably affected by the procedures used for their separation[108]. Contemporary methodologies for separation encompass a range of techniques, such as centrifugation, protein removal, and ultrafiltration[109]. However, there is an elevated risk of protein contamination, and the challenging process might lead to low production rates and high costs[110]. Added to that, there is still scope for enhancement in the field of EV loading technology. The technique of incubating cargo with EVs or cells that secrete EVs is a relatively simple and non-invasive method for passive uptake[111]. Yet it should be noted that the loading capacity of this approach is quite low. Various external techniques, such as electroporation and ultrasonic treatment, have the potential to enhance loading efficiency. Nevertheless, these methods inherently pose a threat of inflicting damage to the products or exosomes that are involved. Thirdly, the connection between EVs and the BBB endures poorly understood[112]. Additional improvements can be implemented to these systems by acquiring a more comprehensive understanding of possible uses of EVs in the field of pharmaceutical transportation.
Additionally, when using EVs as drug carriers to cross the BBB, there may be potential side effects and challenges. Although EVs are generally considered to have low immunogenicity and high biocompatibility, they can still cause adverse immune reactions, such as allergic reactions, cytokine release syndrome, cross-reactivity with endogenous proteins, and non-acute immune responses. EVs may accumulate in non-target cells, causing non-specific toxicity. Unmodified EVs may be rapidly cleared from the bloodstream, leading to low accumulation in target tissues and cells. For long-term use of EVs, their safety over time must be considered, including potential carcinogenicity and other chronic side effects. The ability of EVs to cross the BBB also means that they might spread harmful components to other neurons, thereby exacerbating neurological dysfunction.
4.2.5 Viral vectors
Viral vectors have enhanced gene delivery capabilities, improved delivery efficiency, and reduced cellular damage in comparison to non-viral vectors, thus establishing themselves as a fundamental component in the field of gene therapy. The adeno-associated virus (AAV) is a type of non-enveloped virus that contains single-stranded DNA. AAV exhibits a diminished immune response and has the ability to effectively incorporate exogenous genetic material into multiple host cell types, such as neurons, myocytes, and others[113]. The aforementioned properties render AAV the primary medium for in vivo gene delivery. The primary obstacle in gene therapy for the CNS at present is the development of techniques to effectively penetrate the BBB and deliver target genes. Among the identified wild-type AAVs, serotypes including AAV1, AAV2, AAV4, AAV5, AAV7, AAV8, and AAV9 have demonstrated their ability to effectively transfect neural cells after crossing the BBB via intravenous administration. Yao et al. conducted modifications to the CPP located on the capsid of the wild AAV9 serotype[114]. Through their screening process, they successfully identified a variant of the AAV known as AAV.CPP.16, which showed a notable ability to penetrate the BBB. The development of AAV.CPP.16-aPD-L1-HA, a recombinant AAV construct, was central to the experimental approach. This construct was designed to carry a vector encoding a single chain variable fragment antibody that expresses PD-L1, as well as a gene encoding the hemagglutinin (HA) protein. The AAV.CPP.16-aPD-L1-HA was administered intravenously to a mouse model with fluorescent GL261 glioma. Approximately 26 days following the commencement of the experiment, all mice in the control group without the gene therapy intervention had perished. In contrast, 6 out of 8 mice in the gene therapy group survived for more than 100 days. This outcome suggests that the AAV variant successfully traversed the BBB and established a robust and extensive transfection.
Lentivirus is a type of retrovirus capable of transducing both dividing and non-dividing cells, such as neurons and hematopoietic stem cells. Following the process of reverse transcription, the DNA molecule has the potential to be integrated into the genetic material of the host cell. In contrast to AAVs, lentiviral vectors (LVs) can deliver a larger payload of genetic material, up to 11 kb, to recipient cells[115]. Moreover, the stable integration of transgenic DNA into the genome of the host cells allows for long-term and continuous expression of the transgene in dividing cells. Based on the recent hypothesis that stem cells may cross the BBB, there has been a convergence of brain LV gene therapy and autologous hematopoietic stem cell transplantation. The procedure involves extracting stem cells from the patient's body and subsequently introducing lentivirus encoding therapeutic gene to these cells in a controlled laboratory environment. Subsequently, the modified stem cells are reintroduced into the patient's body through injection. However, whether stem cells can traverse the BBB effectively remains inadequately investigated, and the potential oncogenic consequences of lentivirus-induced insertional mutations are a matter of great concern[116].
It is undeniable that the viral vectors are plagued by several serious problems, including immunogenicity, insertion mutations, and the risk of death after gene delivery[117]. Additionally, the high cost of creating engineered viruses also prohibits their usage.
The above are currently commonly used carriers for penetrating the BBB, and there are many other new carriers that will not be elaborated here.
5. Current Research Challenges and Future Perspectives
In the past two decades, great progress has been achieved in the revelation of the physiology and function of the BBB. We have recognized that the BBB is a well-defined and coordinated regulatory interface that is involved in many activities including transport, secretion, and enzyme release[118]. Whether it is small drugs, peptide or protein drugs, or genes, it is difficult to effectively cross the BBB, which greatly limits the non-invasive therapeutic effects on CNS diseases[119]. We summarize the current research challenges and future perspectives to break through the BBB, as shown in Figure 6. To enhance the efficiency and accuracy of drug delivery, different vehicles specialized for BBB delivery have drawn great interest, where most scientists are concentrating on various nanoparticles, such as polymer nanoparticles, liposomes, dendrimers, exosomes, and viral vectors. The main strategies for these particles are i) escaping the immune system, ii) adopting vehicles that can cross the BBB to improve bioavailability at the targeted site, and iii) having an effective self-navigation function for targeting and/or accumulation enhancement[67]. A successful BBB transport is mostly realized through transcellular pathways or paracellular pathways, however, penetrating the BBB is not sufficient to deliver the drugs to the diseased site. A dual-targeting strategy not only ensures efficient BBB transport, but also utilizes the specific recognition with the highly expressed receptors on the target cells, so as to achieve better delivery effects, which may be a good solution for brain drug delivery in the future. Dual-targeting strategy involves designing and using nanoparticles with two different targeting functions to improve the efficiency and accuracy of drug delivery. On the one hand, by conjugating ligands that can specifically recognize brain endothelial cell receptors (such as transferrin, insulin, folic acid, etc.) to the nanoparticles, the trans-endothelial transport of nanoparticles across the BBB can be facilitated, thereby enhancing the efficiency of drug delivery to the brain. Once the nanoparticles successfully cross the BBB, the second targeting function helps the nanoparticles accumulate in the diseased brain tissue. This is typically achieved by targeting specific receptors or molecules that are highly expressed on the diseased tissue, thereby increasing the concentration of the drug in the affected area. For example, by using phage display peptide library technology, dual-targeting peptides with specific affinity and binding adaptability for brain capillary endothelial cells and cells in brain disease tissue can be selected. These peptides can not only improve the trans-endothelial transport across the BBB but also achieve targeted accumulation in the brain tissue, reducing toxic side effects and offering significant clinical application guidance and reference value. Targeted drug delivery is an interdisciplinary research field involving biotechnology, chemistry, materials science, and medicine. Future research will continue to promote interdisciplinary collaboration to enhance the penetration of the BBB and provide a broader platform for the treatment of brain diseases.
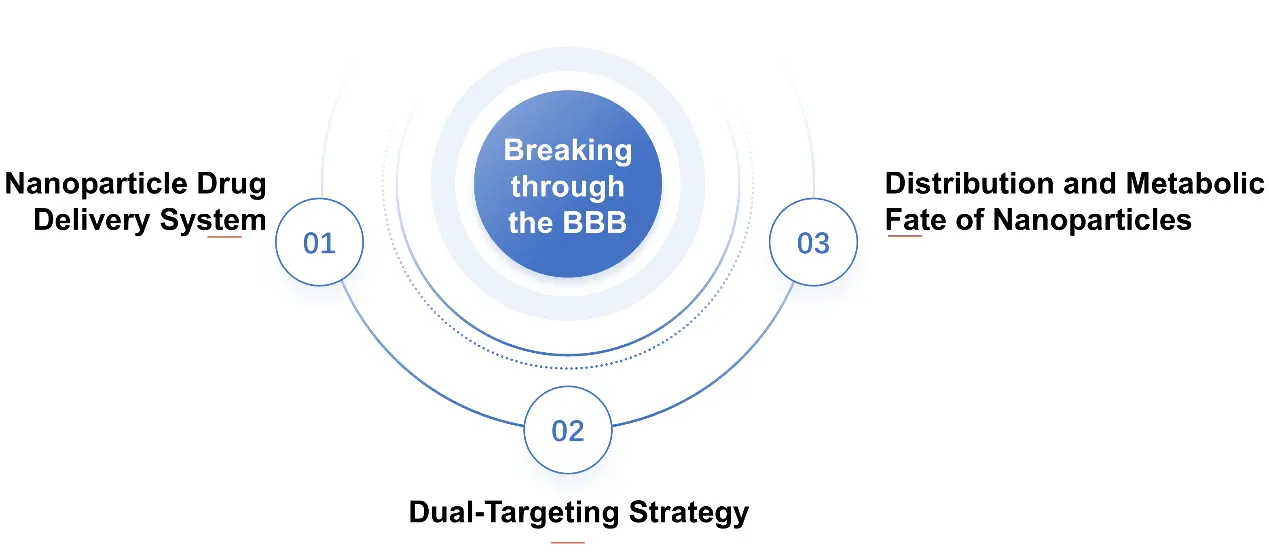
Figure 6. Current research challenges and future perspectives to break through the BBB. BBB: blood-brain barrier.
So far, numerous studies have shown their achievements on high efficiency on brain-targeting, but chronic toxicity evaluation in cellular, organ, tissue, and system levels should engage in further study[8]. Meanwhile, the distribution and metabolic fate of nanoparticles should be evaluated, and the accumulation of these nanomedicine in the brain may make the patient's condition worse. Although most studies have claimed sufficient biocompatibility in their reports, the integrity and function of the BBB after treatment should also be verified, which is pivotal to maintaining the homeostasis of the brain microenvironment.
Author contributions
She P: Writing original draft, reviewing and editing.
Ao X: Writing part of the original draft.
Zhan X, Zhou W: Formal analysis.
Cheng Y, Yang J, Wu S: Resource, data curation.
Zhang X, Peng W: Visualization.
Wang J: Supervision, writing-review & editing, funding acquisition, formal analysis, conceptualization.
Conflicts of interest
The authors declare that there are no conflicts of interest.
Ethical approval
Not applicable
Consent to participate
Not applicable.
Consent for publication
Not applicable.
Availability of data and materials
Not applicable.
Funding
The research was supported by the Natural Science Foundation of Sichuan Province (2023NSFSC1000) and the National Natural Science Foundation of China (52103177).
Copyright
© The Author(s) 2024.
References
-
1. Markowicz-Piasecka M, Markiewicz A, Darłak P, Sikora J, Adla SK, Bagina S, et al. Current chemical, biological, and physiological views in the development of successful brain-targeted pharmaceutics. Neurotherapeutics. 2022;19(3):942-976.
[DOI] -
2. Abbott NJ, Patabendige AA, Dolman DE, Yusof SR, Begley DJ. Structure and function of the blood-brain barrier. Neurobiol Dis. 2010;37(1):13-25.
[DOI] -
3. Barua S, Mitragotri S. Challenges associated with penetration of nanoparticles across cell and tissue barriers: a review of current status and future prospects. Nano Today. 2014;9(2):223-243.
[DOI] -
4. Huang Q, Chen Y, Zhang W, Xia X, Li H, Qin M, et al. Nanotechnology for enhanced nose-to-brain drug delivery in treating neurological diseases. J Control Release. 2024;366:519-534.
[DOI] -
5. Zhang S, Gan L, Cao F, Wang H, Gong P, Ma C, et al. The barrier and interface mechanisms of the brain barrier, and brain drug delivery. Brain Res Bull. 2022;190:69-83.
[DOI] -
6. Zhao Y, Gan L, Ren L, Lin Y, Ma C, Lin X. Factors influencing the blood-brain barrier permeability. Brain Res. 2022;1788:147937-147937.
[DOI] -
7. Hwang SR, Kim K. Nano-enabled delivery systems across the blood-brain barrier. Arch Pharm Res. 2014;37(1):24-30.
[DOI] -
8. Asimakidou E, Tan JKS, Zeng J, Lo CH. Blood-brain barrier-targeting nanoparticles: biomaterial properties and biomedical applications in translational neuroscience. Pharmaceuticals. 2024;17(5):612-612.
[DOI] -
9. Wu D, Chen Q, Chen X, Han F, Chen Z, Wang Y. The blood-brain barrier: structure, regulation, and drug delivery. Sig Transduct Target Ther. 2023;8(1):217-217.
[DOI] -
10. Pardridge WM. Blood-brain barrier delivery. Drug Discov Today. 2007;12(1-2):54-61.
[DOI] -
11. Kadry H, Noorani B, Cucullo L. A blood-brain barrier overview on structure, function, impairment, and biomarkers of integrity. Fluids Barriers CNS. 2020;17(1):69-69.
[DOI] -
12. Wood CAP, Zhang J, Aydin D, Xu Y, Andreone BJ, Langen UH, et al. Structure and mechanism of blood-brain-barrier lipid transporter MFSD2A. Nature. 2021;596(7872):444-448.
[DOI] -
13. Banks WA. From blood-brain barrier to blood-brain interface: new opportunities for CNS drug delivery. Nat Rev Drug Discov. 2016;15(4):275-292.
[DOI] -
14. Pardridge WM. A historical review of brain drug delivery. Pharmaceutics. 2022;14(6):1283-1283.
[DOI] -
15. Iancu G, Serban D, Badiu CD, Tanasescu C, Tudosie MS, Tudor C, et al. Tyrosine kinase inhibitors in breast cancer (Review). Exp Ther Med. 2022;23(2):114-114.
[DOI] -
16. Schlam I, Swain SM. HER2-positive breast cancer and tyrosine kinase inhibitors: the time is now. NPJ Breast Cancer. 2021;7(1):56-56.
[DOI] -
17. Nielsen DL, Andersson M, Kamby C. HER2-targeted therapy in breast cancer. Monoclonal antibodies and tyrosine kinase inhibitors. Cancer Treat Rev. 2009;35(2):121-136.
[DOI] -
18. Xiong B, Wang Y, Chen Y, Xing S, Liao Q, Chen Y, et al. Strategies for structural modification of small molecules to improve blood-brain barrier penetration: a recent perspective. J Med Chem. 2021;64(18):13152-13173.
[DOI] -
19. Hawkins BT, Davis TP. The blood-brain barrier/neurovascular unit in health and disease. Pharmacol Rev. 2005;57(2):173-185.
[DOI] -
20. Ballabh P, Braun A, Nedergaard M. The blood-brain barrier: an overview: structure, regulation, and clinical implications. Neurobiol Dis. 2004;16(1):1-13.
[DOI] -
21. Pardridge WM. Drug transport across the blood-brain barrier. J Cereb Blood Flow Metab. 2012;32(11):1959-1972.
[DOI] -
22. Mikitsh JL, Chacko AM. Pathways for small molecule delivery to the central nervous system across the blood-brain barrier. Perspect Medicin Chem. 2014;6:11-24.
[DOI] -
23. Oldendorf WH, Hyman S, Braun L, Oldendorf SZ. Blood-brain barrier: penetration of morphine, codeine, heroin, and methadone after carotid injection. Science. 1972;178(4064):984-946.
[DOI] -
24. Yi B, Jahangir A, Evans AK, Briggs D, Ravina K, Ernest J, et al. Discovery of novel brain permeable and G protein-biased beta-1 adrenergic receptor partial agonists for the treatment of neurocognitive disorders. PLoS One. 2017;12(7):e0180319-e0180319.
[DOI] -
25. Agrawal M, Saraf S, Saraf S, Dubey SK, Puri A, Patel RJ, et al. Recent strategies and advances in the fabrication of nano lipid carriers and their application towards brain targeting. J Control Release. 2020;321:372-415.
[DOI] -
26. Pardridge WM. Blood-brain barrier and delivery of protein and gene therapeutics to brain. Front Aging Neurosci. 2020;11:373-373.
[DOI] -
27. Winkler EA, Nishida Y, Sagare AP, Rege SV, Bell RD, Perlmutter D, et al. GLUT1 reductions exacerbate Alzheimer's disease vasculo-neuronal dysfunction and degeneration. Nat Neurosci. 2015;18(4):521-530.
[DOI] -
28. Anraku Y, Kuwahara H, Fukusato Y, Mizoguchi A, Ishii T, Nitta K, et al. Glycaemic control boosts glucosylated nanocarrier crossing the BBB into the brain. Nat Commun. 2017;8(1):1001-1001.
[DOI] -
29. Zhao Y, Zhang L, Peng Y, Yue Q, Hai L, Guo L, et al. GLUT1-mediated venlafaxine-thiamine disulfide system-glucose conjugates with "lock-in" function for central nervous system delivery. Chem Biol Drug Des. 2018;91(3):707-716.
[DOI] -
30. Wu KC, Lee CY, Chou FY, Chern Y, Lin CJ. Deletion of equilibrative nucleoside transporter-2 protects against lipopolysaccharide-induced neuroinflammation and blood-brain barrier dysfunction in mice. Brain Behav Immun. 2020;84:59-71.
[DOI] -
31. Kanai Y. Amino acid transporter LAT1 (SLC7A5) as a molecular target for cancer diagnosis and therapeutics. Pharmacol Ther. 2022;230:107964-107964.
[DOI] -
32. Schwarze SR, Hruska KA, Dowdy SF. Protein transduction: unrestricted delivery into all cells? Trends Cell Biol. 2000;10(7):290-295.
[DOI] -
33. Adessi C, Soto C. Converting a peptide into a drug: strategies to improve stability and bioavailability. Curr Med Chem. 2002;9(9):963-978.
[DOI] -
34. Galstyan A, Markman JL, Shatalova ES, Chiechi A, Korman AJ, Patil R, et al. Blood-brain barrier permeable nano immunoconjugates induce local immune responses for glioma therapy. Nat Commun. 2019;10(1):3850-3850.
[DOI] -
35. Guerreiro R, Wojtas A, Bras J, Carrasquillo M, Rogaeva E, Majounie E, et al;. TREM2 variants in Alzheimer's disease. N Engl J Med. 2013;368(2):117-127.
[DOI] -
36. van Lengerich B, Zhan L, Xia D, Chan D, Joy D, Park JI, et al. A TREM2-activating antibody with a blood-brain barrier transport vehicle enhances microglial metabolism in Alzheimer's disease models. Nat Neurosci. 2023;26(3):416-429.
[DOI] -
37. Gonzalez-Rodriguez P, Zampese E, Stout KA, Guzman JN, Ilijic E, Yang B, et al. Disruption of mitochondrial complex I induces progressive parkinsonism. Nature. 2021;599(7886):650-656.
[DOI] -
38. Smajic S, Prada-Medina CA, Landoulsi Z, Ghelfi J, Delcambre S, Dietrich C, et al. Single-cell sequencing of human midbrain reveals glial activation and a Parkinson-specific neuronal state. Brain. 2022;145(3):964-978.
[DOI] -
39. Burbulla LF, Song P, Mazzulli JR, Zampese E, Wong YC, Jeon S, et al. Dopamine oxidation mediates mitochondrial and lysosomal dysfunction in Parkinson's disease. Science. 2017;357(6357):1255-1261.
[DOI] -
40. Lajoie JM, Shusta EV. Targeting receptor-mediated transport for delivery of biologics across the blood-brain barrier. Annu Rev Pharmacol Toxicol. 2015;55:613-631.
[DOI] -
41. Johnsen KB, Burkhart A, Thomsen LB, Andresen TL, Moos T. Targeting the transferrin receptor for brain drug delivery. Prog Neurobiol. 2019;181:101665-101665.
[DOI] -
42. Ramalho MJ, Loureiro JA, Coelho MAN, Pereira MC. Transferrin receptor-targeted nanocarriers: overcoming barriers to treat glioblastoma. Pharmaceutics. 2022;14(2):279-279.
[DOI] -
43. Kawak P, Sawaftah NMA, Pitt WG, Husseini GA. Transferrin-targeted liposomes in glioblastoma therapy: a review. Int J Mol Sci. 2023;24(17):13262-13262.
[DOI] -
44. Choudhury H, Pandey M, Chin PX, Phang YL, Cheah JY, Ooi SC, et al. Transferrin receptors-targeting nanocarriers for efficient targeted delivery and transcytosis of drugs into the brain tumors: a review of recent advancements and emerging trends. Drug Deliv Transl Res. 2018;8(5):1545-1563.
[DOI] -
45. Tang W, Fan W, Lau J, Deng L, Shen Z, Chen X. Emerging blood-brain-barrier-crossing nanotechnology for brain cancer theranostics. Chem Soc Rev. 2019;48(11):2967-3014.
[DOI] -
46. Yang J, Ou W, Jagadeesan N, Simanauskaite J, Sun J, Castellanos D, et al. The effects of a blood-brain barrier penetrating erythropoietin in a mouse model of tauopathy. Pharmaceuticals. 2023;16(4):558-558.
[DOI] -
47. Zhou QH, Boado RJ, Lu JZ, Hui EK, Pardridge WM. Re-engineering erythropoietin as an IgG fusion protein that penetrates the blood-brain barrier in the mouse. Mol Pharm. 2010;7(6):2148-2155.
[DOI] -
48. Kariolis MS, Wells RC, Getz JA, Kwan W, Mahon CS, Tong R, et al. Brain delivery of therapeutic proteins using an Fc fragment blood-brain barrier transport vehicle in mice and monkeys. Sci Transl Med. 2020;12(545):eaay1359-eaay1359.
[DOI] -
49. Gusarova VD, Smolov MA, Lyagoskin IV, Degterev MB, Rechetnik EV, Rodionov AV, et al. Characterization of a HIR-Fab-IDS, novel iduronate 2-sulfatase fusion protein for the treatment of neuropathic mucopolysaccharidosis Type II (hunter syndrome). Bio Drugs. 2023;37(3):375-395.
[DOI] -
50. Lu W. Adsorptive-mediated brain delivery systems. Curr Pharm Biotechnol. 2012;13(12):2340-2348.
[DOI] -
51. Shimon-Hophy M, Wadhwani KC, Chandrasekaran K, Larson D, Smith QR, Rapoport SI. Regional blood-brain barrier transport of cationized bovine serum albumin in awake rats. Am J Physiol. 1991;261(2):R478-R483.
[DOI] -
52. Bickel U, Lee VM, Trojanowski JQ, Pardridge WM. Development and in vitro characterization of a cationized monoclonal antibody against beta A4 protein: a potential probe for Alzheimer's disease. Bioconjug Chem. 1994;5(2):119-125.
[DOI] -
53. Jensen SA, Day ES, Ko CH, Hurley LA, Luciano JP, Kouri FM, et al. Spherical nucleic acid nanoparticle conjugates as an RNAi-based therapy for glioblastoma. Sci Transl Med. 2013;5(209):209ra152-209ra152.
[DOI] -
54. Yurek DM, Fletcher AM, Smith GM, Seroogy KB, Ziady AG, Molter J, et al. Long-term transgene expression in the central nervous system using DNA nanoparticles. Mol Ther. 2009;17(4):641-650.
[DOI] -
55. Kumthekar P, Ko CH, Paunesku T, Dixit K, Sonabend AM, Bloch O, et al. A first-in-human phase 0 clinical study of RNA interference-based spherical nucleic acids in patients with recurrent glioblastoma. Sci Transl Med. 2021;13(584):eabb3945-eabb3945.
[DOI] -
56. Terstappen GC, Meyer AH, Bell RD, Zhang WD. Strategies for delivering therapeutics across the blood-brain barrier. Nat Rev Drug Discov. 2021;20:362-383.
[DOI] -
57. Aly AE, Sun T, Zhang Y, Li Z, Kyada M, Ma Q, et al. Focused ultrasound enhances transgene expression of intranasal hGDNF DNA nanoparticles in the sonicated brain regions. J Control Release. 2023;358:498-509.
[DOI] -
58. Zha S, Liu H, Li H, Li H, Wong KL, All AH. Functionalized nanomaterials capable of crossing the blood-brain barrier. ACS Nano. 2024;18(3):1820-1845.
[DOI] -
59. Song X, Qian H, Yu Y. Nanoparticles mediated the diagnosis and therapy of glioblastoma: bypass or cross the blood-brain barrier. Small. 2023;19:2302613-2302613.
[DOI] -
60. Mei T, Zhang P, Hu Y, Xiao L, Hou J, Nagasaki Y. Engineering hirudin encapsulation in pH-responsive antioxidant nanoparticles for therapeutic efficacy in ischemic stroke model mice. Biomaterials. 2025;314:122860-122860.
[DOI] -
61. Song N, Lu M, Liu J, Lin M, Shangguan P, Wang J, et al. A giant heterometallic polyoxometalate nanocluster for enhanced brain-targeted glioma therapy. Angew Chem Int Ed Engl. 2024;63(10):e202319700-e202319700.
[DOI] -
62. Aly AE, Harmon BT, Padegimas L, Sesenoglu-Laird O, Cooper MJ, Waszczak BL. Intranasal delivery of pGDNF DNA nanoparticles provides neuroprotection in the rat 6-Hydroxydopamine model of parkinson's disease. Mol Neurobiol. 2019;56(1):688-701.
[DOI] -
63. Xie J, Gonzalez-Carter D, Tockary TA, Nakamura N, Xue Y, Nakakido M, et al. Dual-sensitive nanomicelles enhancing systemic delivery of therapeutically active antibodies specifically into the brain. ACS Nano. 2020;14(6):6729-6742.
[DOI] -
64. Teleanu DM, Chircov C, Grumezescu AM, Volceanov A, Teleanu RI. Blood-brain delivery methods using nanotechnology. Pharmaceutics. 2018;10(4):269-269.
[DOI] -
65. Zhou Y, Peng Z, Seven ES, Leblanc RM. Crossing the blood-brain barrier with nanoparticles. J Control Release. 2018;270:290-303.
[DOI] -
66. Zhang Q, Yang L, Zheng Y, Wu X, Chen X, Fei F, et al. Electro-responsive micelle-based universal drug delivery system for on-demand therapy in epilepsy. J Control Release. 2023;360:759-771.
[DOI] -
67. Martins C, Araújo M, Malfanti A, Pacheco C, Smith SJ, Ucakar B, et al. Stimuli-responsive multifunctional nanomedicine for enhanced glioblastoma chemotherapy augments multistage blood-to-brain trafficking and tumor targeting. Small. 2023;19(22):e2300029-e2300029.
[DOI] -
68. Saeedi M, Eslamifar M, Khezri K, Dizaj SM. Applications of nanotechnology in drug delivery to the central nervous system. Biomed Pharmacother. 2019;111:666-675.
[DOI] -
69. Komin A, Bogorad MI, Lin R, Cui H, Searson PC, Hristova K. A peptide for transcellular cargo delivery: structure-function relationship and mechanism of action. J Control Release. 2020;324:633-643.
[DOI] -
70. Xie J, Shen Z, Anraku Y, Kataoka K, Chen X. Nanomaterial-based blood-brain-barrier (BBB) crossing strategies. Biomaterials. 2019;224:119491-119491.
[DOI] -
71. Pang HH, Huang CY, Chou YW, Lin CJ, Zhou ZL, Shiue YL, et al. Bioengineering fluorescent virus-like particle/RNAi nanocomplexes act synergistically with temozolomide to eradicate brain tumors. Nanoscale. 2019;11(17):8102-8109.
[DOI] -
72. Tietz S, Engelhardt B. Brain barriers: crosstalk between complex tight junctions and adherens junctions. J Cell Biol. 2015;209(4):493-506.
[DOI] -
73. Gao X, Qian J, Zheng S, Changyi Y, Zhang J, Ju S, et al. Overcoming the blood-brain barrier for delivering drugs into the brain by using adenosine receptor nanoagonist. ACS Nano. 2014;8(4):3678-3689.
[DOI] -
74. He C, Wu Z, Zhuang M, Li X, Xue S, Xu S, et al. Focused ultrasound-mediated blood-brain barrier opening combined with magnetic targeting cytomembrane based biomimetic microbubbles for glioblastoma therapy. J Nanobiotechnology. 2023;21(1):297-297.
[DOI] -
75. Caraway CA, Gaitsch H, Wicks EE, Kalluri A, Kunadi N, Tyler BM. Polymeric nanoparticles in brain cancer therapy: a review of current approaches. Polymers. 2022;14(14):2963-2963.
[DOI] -
76. Zhang W, Mehta A, Tong Z, Esser L, Voelcker NH. Development of polymeric nanoparticles for blood-brain barrier transfer-strategies and challenges. Adv Sci. 2021;8(10):2003937-2003937.
[DOI] -
77. Rabanel JM, Piec PA, Landri S, Patten SA, Ramassamy C. Transport of PEGylated-PLA nanoparticles across a blood brain barrier model, entry into neuronal cells and in vivo brain bioavailability. J Control Release. 2020;328:679-695.
[DOI] -
78. Hou Q, Zhu L, Wang L, Liu X, Xiao F, Xie Y, et al. Screening on-chip fabricated nanoparticles for penetrating the blood-brain barrier. Nanoscale. 2022;14(8):3234-3241.
[DOI] -
79. Alsaab HO, Alharbi FD, Alhibs AS, Alanazi NB, Alshehri BY, Saleh MA, et al. PLGA-based nanomedicine: history of advancement and development in clinical applications of multiple diseases. Pharmaceutics. 2022;14(12):2728-2728.
[DOI] -
80. Li W, Li B, Wu B, Tian B, Chen X, Wang C, et al. Free-radical cascade generated by AIPH/Fe3O4-coloaded nanoparticles enhances MRI-guided chemo/thermodynamic hypoxic tumor therapy. ACS Appl Mater Interfaces. 2022;14:29563-29576.
[DOI] -
81. Liu HJ, Xu P. Strategies to overcome/penetrate the BBB for systemic nanoparticle delivery to the brain/brain tumor. Adv Drug Deliv Rev. 2022;191:114619-114619.
[DOI] -
82. Li JY, Sabliov C. PLA/PLGA nanoparticles for delivery of drugs across the blood-brain barrier. Nanotechnol Rev. 2013;2:241-257.
[DOI] -
83. Li H, Zha S, Li H, Liu H, Wong KL, All AH. Polymeric dendrimers as nanocarrier vectors for neurotheranostics. Small. 2022;18(45):e2203629-e2203629.
[DOI] -
84. Santos SD, Xavier M, Leite DM, Moreira DA, Custodio B, Torrado M, et al. PAMAM dendrimers: blood-brain barrier transport and neuronal uptake after focal brain ischemia. J Control Release. 2018;291:65-79.
[DOI] -
85. Song C, Ouyang ZJ, Gao Y, Guo HH, Wang SJ, Wang DY, et al. Modular design of multifunctional core-shell tecto dendrimers complexed with copper(II) for MR imaging-guided chemodynamic therapy of orthotopic glioma. Nano Today. 2021;41:101325-101325.
[DOI] -
86. Moscariello P, Ng DYW, Jansen M, Weil T, Luhmann HJ, Hedrich J. Brain delivery of multifunctional dendrimer protein bioconjugates. Adv Sci. 2018;5(5):1700897-1700897.
[DOI] -
87. Liyanage W, Wu T, Kannan S, Kannan RM. Dendrimer-siRNA conjugates for targeted intracellular delivery in glioblastoma animal models. ACS Appl Mater Interfaces. 2022;14(41):46290-46303.
[DOI] -
88. Hersh AM, Alomari S, Tyler BM. Crossing the blood-brain barrier: advances in nanoparticle technology for drug delivery in neuro-oncology. Int J Mol Sci. 2022;23(8):4153-4153.
[DOI] -
89. Kheraldine H, Rachid O, Habib AM, Al Moustafa, Benter IF, Akhtar S. Emerging innate biological properties of nano-drug delivery systems: A focus on PAMAM dendrimers and their clinical potential. Adv Drug Deliv Rev. 2021;178:113908-113908.
[DOI] -
90. Sharma A, Porterfield JE, Smith E, Sharma R, Kannan S, Kannan RM. Effect of mannose targeting of hydroxyl PAMAM dendrimers on cellular and organ biodistribution in a neonatal brain injury model. J Control Release. 2018;283:175-189.
[DOI] -
91. Khan AR, Yang X, Fu M, Zhai G. Recent progress of drug nanoformulations targeting to brain. J Control Release. 2018;291:37-64.
[DOI] -
92. Song XW, Qian HS, Yu YQ. Nanoparticles mediated the diagnosis and therapy of glioblastoma: bypass or cross the blood-brain barrier. Small. 2023;19(45):e2302613-e2302613.
[DOI] -
93. Vieira DB, Gamarra LF. Getting into the brain: liposome-based strategies for effective drug delivery across the blood-brain barrier. Int J Nanomedicine. 2016;11:5381-5414.
[DOI] -
94. Liu S, Liu J, Li H, Mao K, Wang H, Meng X, et al. An optimized ionizable cationic lipid for brain tumor-targeted siRNA delivery and glioblastoma immunotherapy. Biomaterials. 2022;287:121645-121645.
[DOI] -
95. Agrawal M, Ajazuddin , Tripathi DK, Saraf S, Saraf S, Antimisiaris SG, et al. Recent advancements in liposomes targeting strategies to cross blood-brain barrier (BBB) for the treatment of Alzheimer's disease. J Control Release. 2017;260:61-77.
[DOI] -
96. Li LH, Guo QY, Liu YX, Lu MD, Yang J, Ge YL, et al. Targeted combination therapy for glioblastoma by co-delivery of doxorubicin, YAP-siRNA and gold nanorods. J Mater Sci Technol. 2021;63:81-90.
[DOI] -
97. Ismail M, Yang W, Li Y, Chai T, Zhang D, Du Q, et al. Targeted liposomes for combined delivery of artesunate and temozolomide to resistant glioblastoma. Biomaterials. 2022;287:121608-121608.
[DOI] -
98. Karim R, Palazzo C, Evrard B, Piel G. Nanocarriers for the treatment of glioblastoma multiforme: Current state-of-the-art. J Control Release. 2016;227:23-37.
[DOI] -
99. Mohammadinejad R, Dehshahri A, Madamsetty VS, Zahmatkeshan M, Tavakol S, Makvandi P, et al. In vivo gene delivery mediated by non-viral vectors for cancer therapy. J Control Release. 2020;325:249-275.
[DOI] -
100. Qu M, Lin Q, Huang L, Fu Y, Wang L, He S, et al. Dopamine-loaded blood exosomes targeted to brain for better treatment of Parkinson's disease. J Control Release. 2018;287:156-166.
[DOI] -
101. Yang T, Martin P, Fogarty B, Brown A, Schurman K, Phipps R, et al. Exosome delivered anticancer drugs across the blood-brain barrier for brain cancer therapy in Danio rerio. Pharm Res. 2015;32(6):2003-2014.
[DOI] -
102. Hornung S, Dutta S, Bitan G. CNS-derived blood exosomes as a promising source of biomarkers: opportunities and challenges. Front Mol Neurosci. 2020;13:38-38.
[DOI] -
103. Sterzenbach U, Putz U, Low LH, Silke J, Tan SS, Howitt J. Engineered exosomes as vehicles for biologically active proteins. Mol Ther. 2017;25:1269-1278.
[DOI] -
104. Yuan D, Zhao Y, Banks WA, Bullock KM, Haney M, Batrakova E, et al. Macrophage exosomes as natural nanocarriers for protein delivery to inflamed brain. Biomaterials. 2017;142:1-12.
[DOI] -
105. Tian T, Zhang HX, He CP, Fan S, Zhu YL, Qi C, et al. Surface functionalized exosomes as targeted drug delivery vehicles for cerebral ischemia therapy. Biomaterials. 2018;150:137-149.
[DOI] -
106. Kim G, Kim M, Lee Y, Byun JW, Hwang DW, Lee M. Systemic delivery of microRNA-21 antisense oligonucleotides to the brain using T7-peptide decorated exosomes. J Control Release. 2020;317:273-281.
[DOI] -
107. Saeedi S, Israel S, Nagy C, Turecki G. The emerging role of exosomes in mental disorders. Transl Psychiatry. 2019;9:122-122.
[DOI] -
108. Younas N, Fernandez Flores LC, Hopfner F, Höglinger GU, Zerr I. A new paradigm for diagnosis of neurodegenerative diseases: peripheral exosomes of brain origin. Transl Neurodegener. 2022;11(1):28-28.
[DOI] -
109. Banks WA, Sharma P, Bullock KM, Hansen KM, Ludwig N, Whiteside TL. Transport of extracellular vesicles across the blood-brain barrier: brain pharmacokinetics and effects of inflammation. Int J Mol Sci. 2020;21(12):4407-4407.
[DOI] -
110. Perets N, Betzer O, Shapira R, Brenstein S, Angel A, Sadan T, et al. Golden exosomes selectively target brain pathologies in neurodegenerative and neurodevelopmental disorders. Nano Lett. 2019;19(6):3422-3431.
[DOI] -
111. Bashyal S, Thapa C, Lee S. Recent progresses in exosome-based systems for targeted drug delivery to the brain. J Control Release. 2022;348:723-744.
[DOI] -
112. Rufino-Ramos D, Albuquerque PR, Carmona V, Perfeito R, Nobre RJ, Pereira de Almeida L. Extracellular vesicles: novel promising delivery systems for therapy of brain diseases. J Control Release. 2017;262:247-258.
[DOI] -
113. Maguire CA, Ramirez SH, Merkel SF, Sena-Esteves M, Breakefield XO. Gene therapy for the nervous system: challenges and new strategies. Neurotherapeutics. 2014;11(4):817-839.
[DOI] -
114. Yao Y, Wang J, Liu Y, Qu Y, Wang K, Zhang Y, et al. Variants of the adeno-associated virus serotype 9 with enhanced penetration of the blood-brain barrier in rodents and primates. Nat Biomed Eng. 2022;6(11):1257-1271.
[DOI] -
115. McAllister RG, Liu J, Woods MW, Tom SK, Rupar CA, Barr SD. Lentivector integration sites in ependymal cells from a model of metachromatic leukodystrophy: non-B DNA as a new factor influencing integration. Mol Ther Nucleic Acids. 2014;3(8):e187-e187.
[DOI] -
116. Lauer A, Speroni SL, Choi M, Da X, Duncan C, McCarthy S, et al. Hematopoietic stem-cell gene therapy is associated with restored white matter microvascular function in cerebral adrenoleukodystrophy. Nat Commun. 2023;14(1):1900-1900.
[DOI] -
117. Kumar P, Wu H, McBride JL, Jung KE, Kim MH, Davidson BL, et al. Transvascular delivery of small interfering RNA to the central nervous system. Nature. 2007;448(7149):39-43.
[DOI] -
118. Correale J, Villa A. Cellular elements of the blood-brain barrier. Neurochem Res. 2009;34(12):2067-2077.
[DOI] -
119. Lochhead JJ, Yang J, Ronaldson PT, Davis TP. Structure, function, and regulation of the blood-brain barrier tight junction in central nervous system disorders. Front Physiol. 2020;11:914-914.
[DOI]
Copyright
© The Author(s) 2024. This is an Open Access article licensed under a Creative Commons Attribution 4.0 International License (https://creativecommons.org/licenses/by/4.0/), which permits unrestricted use, sharing, adaptation, distribution and reproduction in any medium or format, for any purpose, even commercially, as long as you give appropriate credit to the original author(s) and the source, provide a link to the Creative Commons license, and indicate if changes were made.
Share And Cite