Chaoliang Tan, Department of Electrical Engineering, City University of Hong Kong, Kowloon, Hong Kong SAR 999077, China. E-mail: chaoltan@cityu.edu.hk
Abstract
Circularly polarized light (CPL) features electromagnetic vectors that rotate regularly in a plane perpendicular to the direction of propagation, transmitting optical chirality information that is imperceptible to human beings. CPL can be classified into the left-handed and right-handed circularly polarization light (L-/R-CPL), depending on whether the rotation direction is clockwise or anticlockwise, respectively. The ability to manipulate and characterize CPL is crucial for advancing various optical technologies, making the effective and direct detection of CPL extremely important. Breeding in the hotbed provided by the explosively increased chiral materials with CPL luminescence and strong circular dichroism (CD), CPL detectors are currently experiencing savage growth. Mainstream strategies can be divided into the leverage of photoactive materials with inherent chirality and the integration of chiral metamaterials with nonchiral photoactive materials. In this review, we not only highlight significant material innovations and detector architectures for CPL detection but also address the broader implications of these advancements. We discuss the challenges and future directions in this field, particularly focusing on how these developments could impact existing commodities, such as polarimetric imaging and security communications, and contribute to sustainability in technology through improved detection efficiency. Our goal is to inspire further promising developments in CPL photodetectors and encourage a broader application spectrum.
Keywords
1. Introduction
Light, serving as a high-entropy information carrier, connects human minds to the real world through the eyes. Without exaggeration, modern civilization has been built on the foundation of understanding and manipulating light[1]. In addition to the commonly utilized characteristics of light, such as frequency and intensity, the polarization state of light offers another dimension for developing advanced optical technologies in areas like quantum physics and spectroscopy[2-4]. Polarized light can generally be classified into linearly and elliptically polarized light. Circularly polarized light (CPL), a special case of elliptically polarized light that conveys the spin angular momentum of
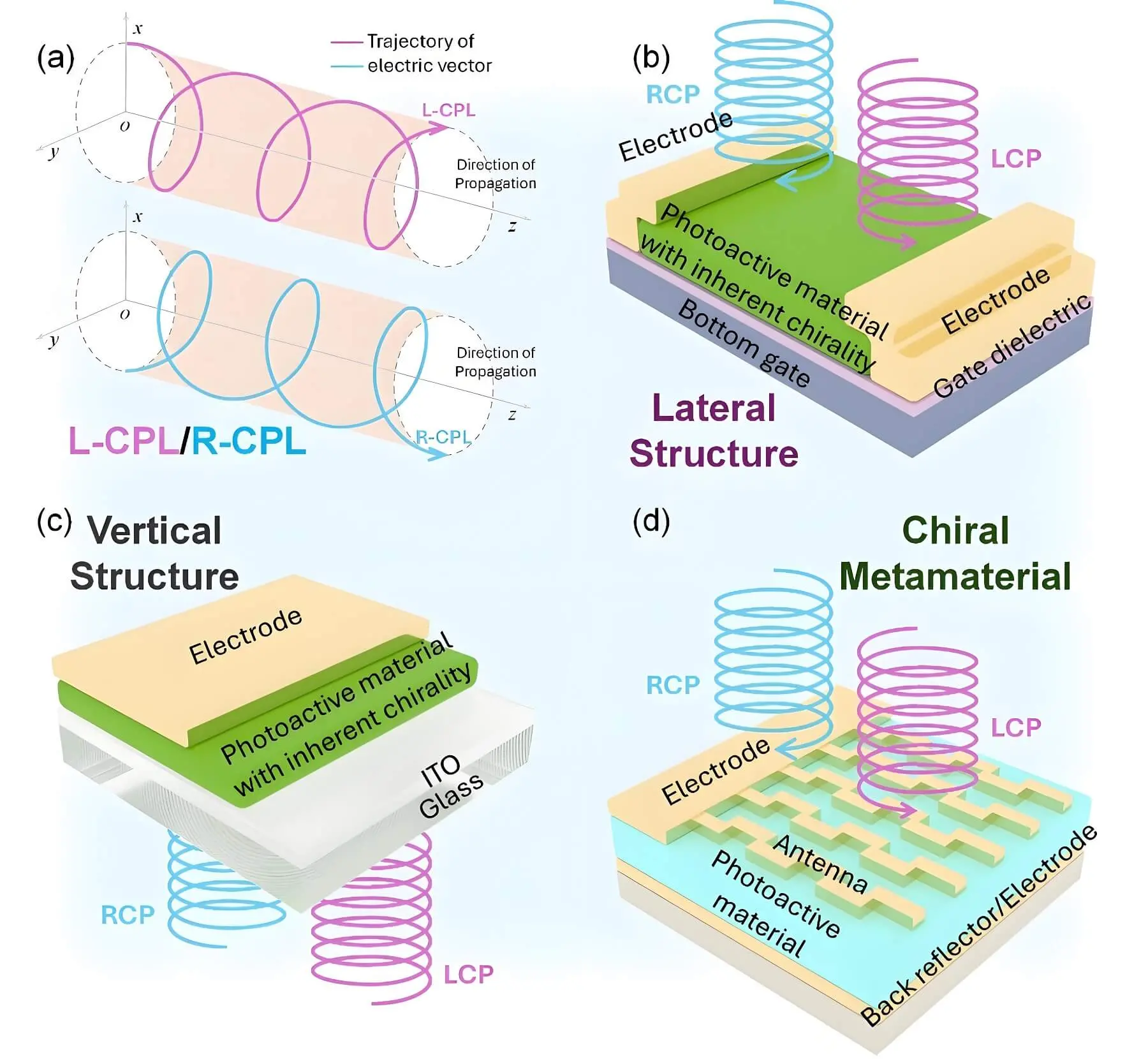
Figure 1. (a) Definition of LCP/RCP light (left/right); Schematic illustrations of (b) lateral; (c) vertical CPL photodetectors based on photoactive materials with inherent chirality; (d) CPL photodetectors enabled by chiral metamaterial. LCP: left-handed circularly polarized; RCP: right-handed circularly polarized; CPL: circularly polarized light; ITO: indium-tin oxide.
Recently, the burgeoning development in the synthesis of various photoactive materials with inherent chirality and the design of chiral metamaterials has significantly alleviated this issue[12-33]. The commonly used chiral materials for CPL detection can be categorized into chiral organic materials, chiral inorganic materials, and chiral organic-inorganic hybrid materials[12,34-36]. By leveraging these materials, researchers have developed CPL photodetectors with architectures that include lateral photoconductors and phototransistors (Figure 1b)[14,19,21,24,36-49], as well as vertical photodiodes (Figure 1c)[16,17,23,50-53]. In addition to using photoactive materials with inherent chirality, researchers have also extensively fabricated CPL photodetectors by patterning chiral metamaterials onto semiconducting channels using micro-/nano-fabrication technologies (Figure 1d)[30,54-59]. Despite these encouraging advancements, the miniaturization of CPL photodetectors still has a long way to go before commercialization can be realized. Many challenges remain to be addressed in this research field.
In this review, we first provide an overview of common chiral materials used for CPL detection, followed by a discussion of various CPL photodetector architectures, with an emphasis on their performance metrics. This is expected to offer valuable guidance for future optimization of device performance. Next, representative and promising application prospects of CPL photodetectors will be reviewed. In the end, we provide an outlook on the challenges within the research field of CPL detection, specifically the issues related to the limited discrimination capability of currently reported CPL photodetectors, the poor thermal stability and small carrier mobilities of most chiral organic materials used for CPL detection, and the fact that the design and fabrication of CPL photodetectors are still confined to the single-device level. The insights presented in this review have the potential to significantly impact fields such as in-sensor neuromorphic computing systems using CPL as the input, polarimetric imaging, and security optical communications, where advanced CPL detection can facilitate substantial advancements.
2. Chiral Materials for CPL Detection
Chiral materials refer to objects that cannot be superimposed onto their mirror images. Due to this unique structure, these materials often exhibit different light-matter interactions with left-handed and right-handed circular polarization states, which enables discrimination between L-CPL and R-CPL. Commonly used chiral materials for CPL detection include chiral organic materials and chiral organic-inorganic hybrid perovskites. Compared to inorganic materials, organic materials typically offer several advantages when applied in photodetection, such as low preparation cost, high mechanical flexibility, large light absorption coefficient, and light weight per volume[60-62]. Moreover, the extensive variety in chemical synthesis pathways allows for the introduction of chirality, which enables CPL sensitivity[63]. Chiral organic materials can usually be categorized into chiral molecules (Figure 2a)[14,19,64], self-assembled supramolecular structures (Figure 2b)[37,39,65], and chiral conjugated polymers (Figure 2c)[13,50,66,67].
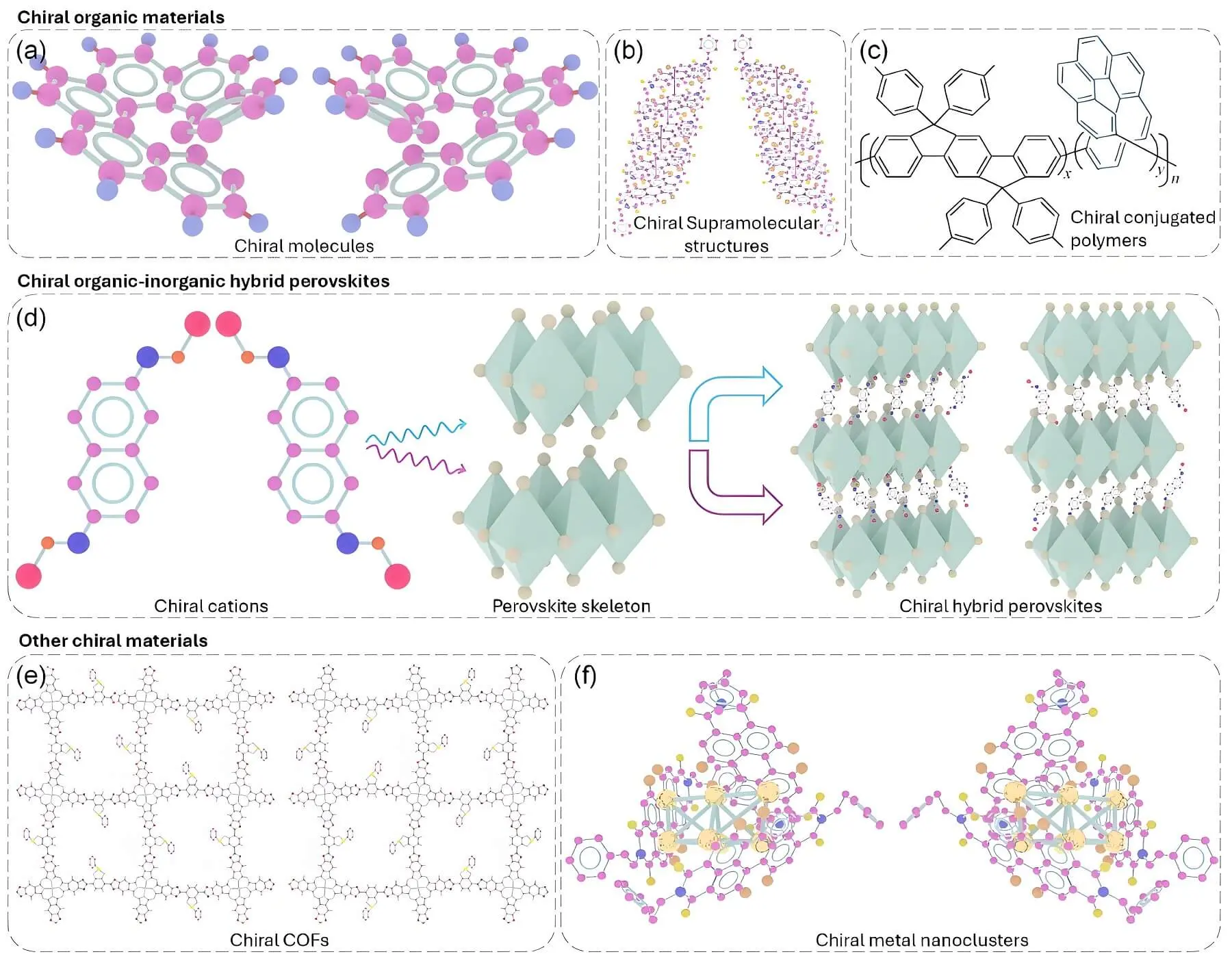
Figure 2. Schematic illustration of chiral materials for CPL detection. (a) Chiral molecules; (b) supramolecular structures; (c) chiral conjugated polymers; (d) chiral organic-inorganic hybrid perovskites. Other chiral materials such as (e) chiral COFs and (f) chiral metal nanoclusters. CPL: circularly polarized light; COFs: covalent-organic frameworks.
Typical examples of chiral molecules are helical-shaped helicenes and chiral fullerenes. Helicenes consist of ortho-condensed aromatic rings, which are angularly conjugated to form a screw-shaped skeleton, as depicted in Figure 2b[68]. Depending on the screw directions, helicenes can be usually found in both right-handed and left-handed forms. This helically chiral molecular structure endows these materials with many intriguing chirality-related responses. In 2023, Yang et al. prepared the 1-aza[6]helicene via a previously reported synthetic strategy, successfully separating the 1-aza[6]helicene into enantiomerically pure right-handed (+)-1-aza[6]helicene and left-handed (-)-1-aza[6]helicene[14,69]. Leveraging the good solubility of 1-aza[6]helicene in toluene, the authors prepared the thin films of (+)-1-aza[6]helicene and (-)-1-aza[6]helicene through spin-coating and demonstrated the strong handedness-dependent optical absorption in the CD spectra. Subsequent phototransistors were demonstrated, which could effectively discriminate between L-CPL and R-CPL thanks to the chiroptical properties of the 1-aza[6]helicene. Beyond organic molecules with helical chirality, other chiral compounds, including axially chiral and point chiral molecules, also demonstrate significant potential for application in CPL detection[70]. In 2024, Kwon et al. synthesized a pair of axially chiral enantiomers, (R)-1 and (S)-1, by linking the 1,1'-binaphthyl (BN) chiral scaffold to the n -type semiconducting naphthalene diimides (NDIs)[71]. They then deposited thin films of both enantiomers via thermal evaporation onto SiO2/n++ Si wafers. These films were utilized to fabricate organic field-effect transistors (OFETs) that exhibited significant CPL discrimination capability. Additionally, point chiral molecules, such as chiral methylbenzylammonium (MBA) molecules, have been widely incorporated into photoactive materials like perovskites for CPL detection, as will be discussed later[38,72,73]. Chiral fullerenes, derivatives of the famous carbon spheres, have also been used to advance CPL detection[74]. Although the physicochemical properties of the fullerenes have been enriched via the development of various derivatives, the poor solubility of the parent fullerene in organic solvents impedes their application prospects. The preparation of bis[60] phenyl-C61-butyric acid methyl ester(PCBM) through the addition of solubilizing addends to C60 using the cycloaddition chemistry has significantly addressed this issue[75,76]. In 2021, Shi et al. separated ten pairs of enantiomers from the nineteen structural isomers of bis[60]PCBM[36]. Based on the comprehensive study of the chiroptical properties of these chiral fullerenes, the authors concluded that greater asymmetry in the electron distribution within the fullerene cage leads to a larger chiroptical response. This finding provides a guideline for designing chiral fullerenes for CPL detection.
Beyond chiral molecules, helical-arranged supramolecular assemblies, which are schematically illustrated in Figure 2b, also exhibit strong chiroptical properties via the chirality amplification effect[77,78]. This is typically achieved via the so-called "sergeants-and-soldiers" and "majority rules", where the chirality of the supramolecular assembles is controlled by a small portion of chiral molecules and a slight enantiomeric excess, respectively[63,79]. In 2017, Shang et al. prepared the homochiral and heterochiral n-type nanowires based on the supramolecular assemblies of N,N'-bis-(1'-phenylethyl)per-ylene-3,4,9,10-tetracarboxyldiimide molecules via the bottom-up self-assembly strategy and systematically characterized their electrical and chiroptical properties[37]. The heterochiral nanowires were fabricated via the alternative self-stacking of opposite enantiomers, while the homochiral nanowires were fabricated from pure enantiomers. Compared to their monomeric equivalents, the helical-arranged supramolecular assemblies exhibited significantly enhanced chiroptical sensing, demonstrating the amplification of chiroptical responses. Aside from these chiral molecular assemblies, achiral compounds can also be used to prepare chiral supramolecular aggregates[70,80,81]. In 2022, Liu et al. reported on the development of small-molecule organic solar cell featuring supramolecular chirality for CPL detection[80]. These devices were constructed from the bulk heterojunction, a blend of chiral small-molecular diketopyrrolopyrrole-hexathiophene as the electron donor with achiral (6,6)-phenyl C61 butyric acid methyl ester as the electron acceptor. Although the helicity of the resulting supramolecular aggregates in films was not clearly discernible, their significant chiroptical properties were confirmed by strong CD signals. This discovery suggests that incorporating achiral components into chiral aggregates do not compromise the formation of the chiral phase.
Conjugated polymers, exhibiting advantages such as facilitated preparation in thin films and high tunability for optoelectronics, can also be designed for CPL detection[66,67]. To induce chiroptical properties of these material systems, several strategies have been developed, including the direct synthesis of inherently chiral polymers and the doping of achiral materials with chiral dopants. For instance, Gilot et al. prepared polymer thin films of chiral poly(fluorene- alt -dithienylbenzothiadiazole) (PFDTMT) 1 with large CD signal for photovoltaic cells sensitive to CPL[13]. Compared to the device fabricated from the achiral analogue PFDTMT 2, which presents a small average dissymmetry factor (gsc = +2 × 10-5) for the photocurrent under the illumination of R-CPL and L-CPL, the photovoltaic cell made from PFDTMT 1 shows a much higher value of +0.7 × 10-2. This significant contrast corroborated the application potential of chiral conjugated polymers for CPL detection. On the other hand, the direct synthesis of chiral polymers can be hindered by the low yields and complex synthesis routes. Alternatively, doping chiral molecules into achiral polymers, as illustrated in Figure 2c, could be another viable strategy. This simple and straightforward approach can indue the transfer of chirality from chiral molecules to semiconducting polymers. In 2019, Kim et al. blended the achiral poly[3-(6-carboxyhexyl)thiophene-2,5-diyl] (P3CT) polymer semiconductor with helical (R)-(+)-1,1'-binaphthyl-2,2'-diamine (BN(R))/(S)-(-)-1,1'-binaphthyl-2,2-diamine (BN(S)) molecules to obtain thin films with chiroptical responses[50]. These responses can be attributed to the intermolecular interactions between P3CT and BN molecules.
In addition to chiral organic materials, the chiral organic-inorganic materials system, represented by the integration of chiral cations into perovskite skeletons, has also been extensively studied for highly efficient and compact CPL detection[21,23,24,40,42,53,82-86]. Perovskites nowadays have been extensively used in photovoltaics due to their superior optoelectronic properties, which include low preparation cost, high light absorption coefficients, tunable absorption spectra[87,88]. Many effective strategies have been proposed to endow perovskites with both high mobility and photosensitivity. In 2022, Ferreira et al. developed a simple salt treatment to improve charge transfer by connecting perovskite quantum dots[89]. This technique significantly optimized the photodetector performance, achieving a high dark hole mobility of 14.2 cm2 V-1 s-1 and a specific detectivity of 1016 Jones. In addition, perovskite compositions are highly tunable, enabling the introduction of chiral organic molecules as cations (left panel in Figure 2d). This synthesis strategy allows for the integration of excellent optoelectronic properties with the chirality, facilitating the development of organic-inorganic hybrid perovskites (right panel in Figure 2d) for CPL detection. In 2019, Chen et al. selected the enantiomers of right-handed α-phenylethylamine (R-α-PEA) and left-handed α-phenylethylamine (S-α-PEA) to prepare the organic-inorganic hybrid perovskite (R- and S-α-PEA)PbI3 [21]. After dissolving the prepared chiral perovskites into N,N-anhydrous dimethylformamide and spin-coating the precursor solution into thin films, the authors observed two intense peaks at 328 and 392 nm in the CD spectra of the films. The strong CD signals suggest the application potential for these chiral perovskites in subsequent CPL photodetectors. Considering the environmental issues associated with leaded perovskites, Li et al. prepared chiral lead-free hybrid perovskites, [(R)-β-MPA]4AgBiI8((R)-β-MPA = (R)-(+)-β-methylphenethylammonium, 1-R) and [(S)-β-MPA]4AgBiI8((S)-β-MPA = (S)-(+)-β-methylphenethylammonium, 1-S)[40]. The thin films of 1-R and 1-S exhibited strong CD signals with opposite values in their CD spectra, corroborating the chiroptical properties of these prepared chiral organic-inorganic hybrid perovskites.
Chiral organic materials and organic-inorganic hybrid perovskites constitute the main body of photoactive materials with inherent chirality for CPL detection. Additionally, chiral covalent-organic frameworks (COFs) (Figure 2e), chiral metal-organic frameworks (MOFs) and other chiral organic-inorganic hybrids such as chiral metal nanoclusters (Figure 2f) also deserve to be explored to advance CPL detection[26-28,38,90-92]. For example, in 2023 and 2024, Gu et al. and Tang et al. demonstrated the construction of two-dimensional (2D) chiral COFs by introducing 5,10,15,20-tetrakis(4-aminophenyl)porphyrinaldehyde to chiral 2,5-bis((S/R)-2-methylbutoxy)terephthalaldehyde and the condensation reactions of C3-symmetric 4,4',4''-(1,3,5-triazine-2,4,6-triyl)tribenzaldehyde (Tz) with three flexible chiral dihydrazide linkers derived from malic acid, aspartic acid and tartaric acid for CPL detection, respectively[26,27]. Other metal frameworks can also be integrated with chiral organic molecules for chiral hybrids. In 2021, Hao et al. reported the synthesis of chiral organic-inorganic hybrids by integrating zero-dimensional (0D) chiral copper chloride templated with chiral methylbenzylammonium ((R-/S-MBA)2CuCl4) for direct CPL detection[38]. The chiroptical properties of the prepared (R-/S-MBA)2CuCl4 were demonstrated by the strong CD signals in their CD spectra. In 2024, He et al. synthesized a pair of enantiomers, silver nanoclusters (R/S-Ag7), through the reaction between organic chiral R/S-2-diphenyl-2-hydroxymethylpyrrolidine-1-dithiocarboxylic acid (R/S-DMA) and 2,6-bis(diphenylphosphino)pyridine (dpppy) in the presence of inorganic salt AgBF4[28].
The vast synthesis routes for abundant chiral materials have significantly advanced the development of CPL photodetectors. However, the chiroptical responses of most materials originate from chiral organic molecules, which may compromise the endurability of the subsequent devices. In the future, more efforts will be needed to optimize device architecture and develop chiral materials with higher thermal stability.
3. Figures of Merit for CPL Photodetectors and Device Architectures
The performance of CPL photodetectors can be evaluated similarly to those traditional photodetectors by figures of merit such as responsivity, external quantum efficiency (EQE), specific detectivity (D*), and response speed, except for the most crucial parameter, the dissymmetry factor (g). This dissymmetry factor measures the ability of CPL photodetectors to distinguish L-CPL and R-CPL. The dissymmetry factor g is typically defined by the photocurrents (IL and IR) under the illumination of L-CPL and R-CPL as[63]
The dissymmetry factor can also be defined using photoresponsivity as gres. It should be noted that gres usually equals gph because it is essential to maintain consistent laser power for both L-CPL and R-CPL during the measurement of the discrimination ability.
Since CPL photodetectors exhibit differentiated light-matter interactions under the illumination of L-CPL and R-CPL, other figures of merit should be characterized with speciating the polarization state of light.
Responsivity, measuring the ability of the CPL photodetector to convert the optical signals into electrical signals, is defined as[93]
where
EQE, telling the additional carriers generated per incident photon, is calculated by
where h, c, e, and λ are Planck's constant, light speed, elementary charge, and the wavelength of the incident light, respectively.
D*, a critical figure of merit for photodetectors, quantifies their ability to detect weak signals while considering the device configurations. It is calculated by
where A represents the photoactive area, and Δf(L) and Δf(R) denote the bandwidths of the photodetectors for L-CPL and R-CPL, respectively. The noise equivalent power for each polarization, NEP(L) and NEP(R) indicate the minimum light signal power that can be distinguished from the background noise under the illumination of left handed circularly polarized (LCP) and right hand circularly polarized (RCP) light, respectively. NEP is defined by the equation,
Here, in is the background noise current, which simplifies to
Response time (τr) measures the response speed of the photodetector to optical signals and typically consists of two components: rise time (τrise) and fall time (τfall). τrise is defined as the time required for the photocurrent in the device to increase from 10% to 90% of its peak value, while τfall represents the time it takes to decrease from 90% to 10%. Most reported CPL photodetectors present slow response speeds, limited by the small carrier mobilities in organic thin films.
Leveraging the chiroptical response of photoactive materials with inherent chirality, CPL photodetectors can adopt either lateral (Figure 1b) or vertical (Figure 1c) structures to maximize the interactions between CPL-dipole (or charge) interactions within these materials[14,21,30,94-96]. Lateral-structure CPL photodetectors usually include phototransistors and photoconductors. Compared to photoconductors, phototransistors are equipped with a third gate electrode that tunes the carrier densities via an applied vertical electrical field[97,98]. A phototransistor can also operate in the photoconductor mode without applying gate voltage. Figure 3a illustrates a CPL phototransistor, as reported by Lee et al., constructed from a heterostructure composed of chiral of 2,2'-((4,4'-((2,5-bis(2-octyldodecyl)-3,6-dioxo-2,3,5,6-tetrahydropyrrolo[3,4-c]pyrrole-1,4-diyl)bias(thiophene-5,2-diyl))bis(benzoyl))bis(azanediyl))bis(N1,N5-didodecylpentanediamide) (DPPPT) nanohelices and InGaZnO (IGZO)[44]. Utilizing the considerable chiroptical properties of the synthesized chiral nanohelices, the right-handed CPL phototransistor (R-DPPPT-cPTr) demonstrated effective discrimination between 520-nm L-CPL and R-CPL, as depicted in Figure 3b. In addition, following the successful preparation of right-handed (+)-1-aza[6]helicene and left-handed (-)-1-aza[6]helicene thin films, Yang et al. proceeded to fabricate phototransistors for direct CPL detection[14]. The demonstrated organic transistor exhibited a considerable on/off current ratio of ~ 104 at a bias of Vds = -60 V in the dark. The extracted field-effect mobility reached μFE = 10-4 cm2 V-1 s-1. Moreover, based on the chiroptical responses of the prepared chiral thin films, the fabricated helicene phototransistors exhibited distinct response to CPL with different polarization states. The transfer curves for the device based on right-handed (+)-1-aza[6]helicene thin film were measured in the dark and under illumination of L-CPL and R-CPL, respectively. The wavelength of the CPL was set at 365 nm, at which these helicene thin films exhibit the strongest CD signals. This result demonstrates that chiral (+)-1-aza[6]helicene molecules give this phototransistor a stronger response to R-CPL than to L-CPL. It is worth mentioning that the freshly spin-coated helicene films underwent an annealing process to increase the size of the crystal domains, a common strategy employed to enhance the quality of organic thin films[99]. Similarly, based on the preparation of chiral thin films containing organic-inorganic hybrid perovskites (R- and S-α-PEA)PbI3, Chen et al. reported on the fabrication of flexible CPL photoconductors. The photograph of the fabricated device is shown in the inset of Figure 3c, where flexible polyethylene terephthalate was selected as the substrate. Compared with using the rigid quartz as the substrate, the flexible CPL photoconductor achieves a slightly smaller dissymmetry factor of gres = 0.08 < 0.1. The responsivity of the flexible device under 395-nm LCP and 395-nm RCP are 0.090 and 0.098 A W-1, respectively. Moreover, after bending 100 times, gres did not show any observable degradation, as shown in Figure 3c.
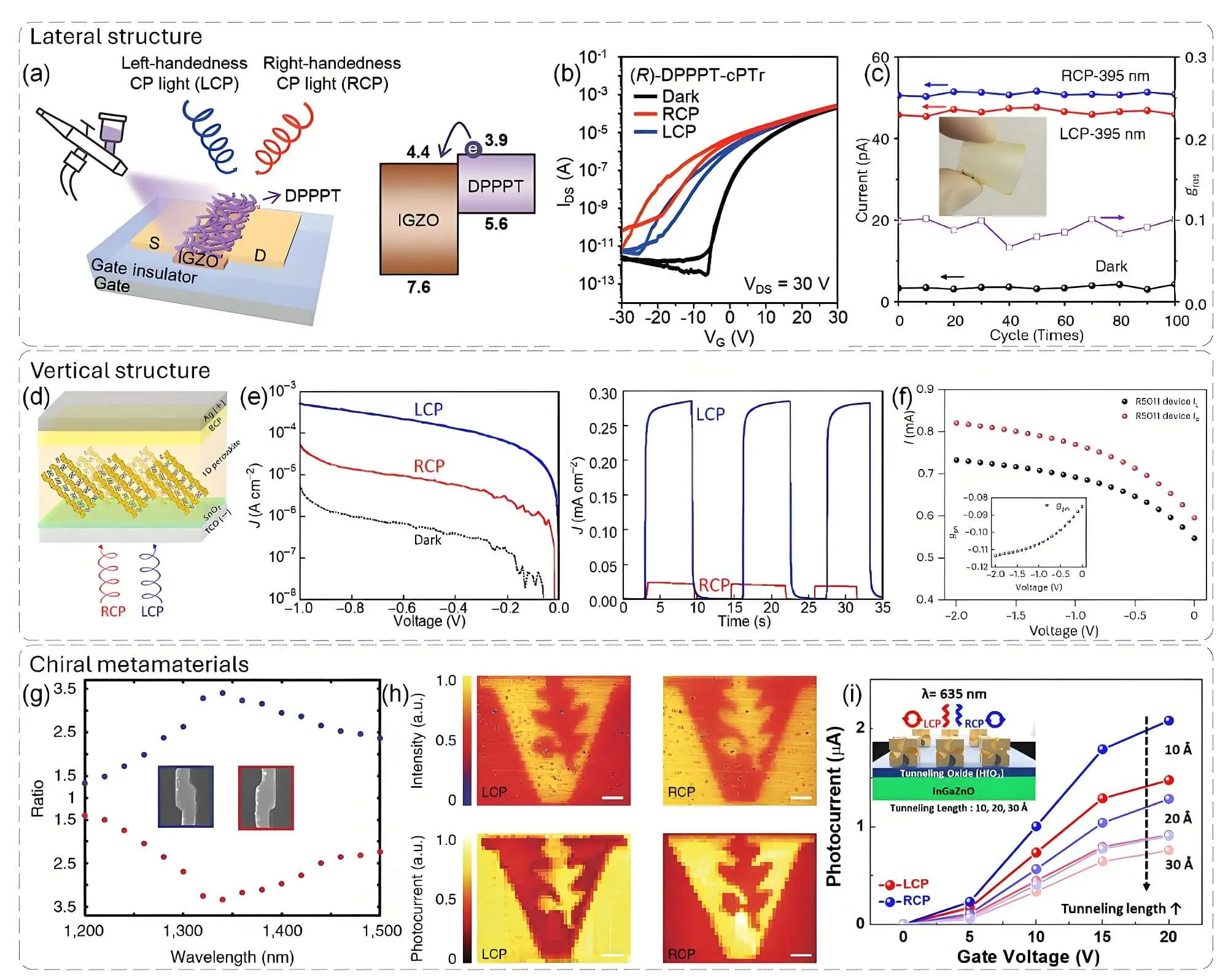
Figure 3. Later-structure CPL photodetectors. (a) Schematic illustration of a CPL phototransistor based on a heterojunction comprising DPPPT nanohelices and IGZO; (b) transfer curves of (R)-DPPPT CPL phototransistor measured in the dark, and under illumination of LCP and RCP light, respectively. Vds = 30 V, λ = 520 nm, Pin = 500 μW cm-2. Republished with permission from[44]; (c) folding cycles-dependent current variation of the CPL photoconductor made from (R-α-PEA)PbI3, measured in the dark and under illumination of 395-nm L-CPL, R-CPL, respectively, along with gres. Inset provides the photograph of the fabricated device[21]. Vertical-structure CPL photodetectors: (d) schematic illustration of a photodiode made from the helical 1D (R-/S-NEA)PbI3 perovskite; (e) left panel: J-V curves of the photodiode made from (R-NEA)PbI3 measured in the dark, and under illumination of LCP and RCP light, respectively. Right panel: Current evolution triggered by LCP and RCP light pulses, respectively. V = -0.5 V, λ = 395 nm, Pin = 1.0 mW cm-2. Republished with permission from[23]; (f) current-voltage curves of the CPL photodiode based on chiral ITIC blends under illumination of L-CPL and R-CPL. Inset: Voltage-dependent gph[96]. CPL photodetectors enabled by chiral metamaterials: (g) wavelength-dependent CPL discrimination ratio of the device decorated by left-handed and right-handed metamaterials, respectively. Inset provides the SEM image of the prepared metamaterials; (h) images of the Vanderbilt University logo, patterned with left-handed and right-handed metamaterial under illumination of L-CPL (upper left) and R-CPL (upper right). Photocurrent mappings of the metamaterial pattern under illumination of L-CPL (lower left) and R-CPL (lower right). Scale bar: 10 μm[30]; (i) gate voltage-dependent photocurrent of the phototransistor, decorated by chiral Au nanoparticles, under illumination of L-CPL and R-CPL. Inset depicts the schematic illustration of the device and measurement conditions[101]. IGZO: InGaZnO; DPPPT: 2,2'-((4,4'-((2,5-bis(2-octyldodecyl)-3,6-dioxo-2,3,5,6-tetrahydropyrrolo[3,4-c]pyrrole-1,4-diyl)bias(thiophene-5,2-diyl))bis(benzoyl))bis(azanediyl))bis(N1,N5-didodecylpentanediamide); CPL: circularly polarized light; R-α-PEA: right-handed α-phenylethylamine; SEM: scanning electron microscopy.
Vertical-structure CPL photodetectors primarily comprise photodiodes, with transparent indium-tin oxide (ITO) commonly used as the electrode to facilitate the reception of optical signals[95,96]. A unique advantage of photodiode compared to phototransistors and photoconductors is that photodiodes can separate photogenerated carriers in built-in electric fields, enabling self-powered photodetection without the need for an external electric bias[73,100]. Figure 3d illustrates a photodiode made from one-dimensional chiral perovskite R-(+)- and S-(-)-1-(1-naphtyl)ethylamine (R- and S-NEA)PbI3 [23]. In this configuration, the transparent conducting oxide was selected as the bottom electrode to facilitate the incidence of L-CPL and R-CPL. As shown in Figure 3e, the fabricated device exhibited superior discrimination capability between 395-nm L-CPL and R-CPL. In 2024, Zhang et al. demonstrated self-powered CPL photodiodes using 2D chiral perovskites[73]. These perovskites incorporated mixed chiral aryl (R)-(+), (S)-(-)-α-methylbenzylammonium and achiral alkyl n-butylammonium cations. In the design of their vertical photodiode, the built-in potential, facilitated by an asymmetrical electrode configuration, enables the separation of photogenerated carriers without the need for an external bias voltage. This design allows the device to operate with self-powered capabilities, achieving a specific detectivity of 2.45 × 1012 Jones at zero bias. In 2023, Song et al. reported on CPL photodetection based on helical polymers[95]. The helical photoactive materials were synthesized using a chiral templating methodology. Which involved the use of a volatile enantiomer (R5011/S5011) as the growth template, which was later removed via sublimation. Additionally, R[6,6]-phenyl-C71-butyric acid methyl ester (PC70 BM) was incorporated as the charge acceptor unit in the device. Based on these prepared flexible chiroptical layers, the authors demonstrated effective CPL detectionand the reported CPL photodetector successfully monitored the polarization state of the incident CPL, achieving an excellent dissymmetric factor gph = -1.2. According to the authors, this is the highest value among organic photodiodes fabricated from continuous and homogenous active layers. The authors further extended the photodiodes into a 30 × 30 matrix covering a 3 × 3 cm2 area, which clearly captured a 'heart-shaped' image in CPL detection mode. Limited by the large bandgap of the chiral organic materials, the response range of the demonstrated CPL photodetectors is typically confined to the ultraviolet to visible (UV-vis) spectra. In 2023, Wan et al. developed a strategy that enabled CPL detection in the near infrared (NIR) regime[96]. Their strategy, demonstrated to be effective with various non-fullerene acceptors (NFAs), leveraged the strong excitonic coupling between adjacent NFA molecules to introduce strong CD signals in NFAs-based thin films. In experiments, the archetypal NFA, a symmetric indacenodithieno[3,2-b]-thiophene (IDTT) core and two 1,1-dicyanomethylene-3-indanone (IC) end groups, was selected to blend with the chiral compound R5011 to fabricate a CPL photodiode, as shown in Figure 3f. This photodiode exhibited strong discrimination capability towards 700-nm L-CPL and R-CPL, achieving a maximum gph = -0.11 at a bias of -2.0 V (Figure 3f). The authors attributed this NIR response to the strong light absorption in that band, induced by a combination of the π-π* IDTT transition and a charge transfer transition from the IDTT core to the IC end.
Beyond lateral-structure and vertical-structure CPL photodetectors that utilize inherently chiral photoactive materials, chiral metal nanostructures can also be designed to impart chiroptical responses to achiral photoactive materials[30,55,101]. These chiroptical responses are mainly related to the generation of hot carriers in chiral metamaterials under CPL illumination, and the response spectra typically depend on the geometry design of the metamaterials[102,103]. Different from those photodetectors based on photoactive materials with inherent chirality, which mainly exhibit chiroptical response in the UV-vis range, these devices enabled by chiral metamaterials demonstrate a longer wavelength response. This response is determined by the geometrical sizes of the metamaterials[96]. In 2015, Li et al. specifically designed chiral plasmonic metamaterials beneath n-type silicon (Si) to create an ultracompact CPL detector[30]. The chiral metamaterials have been patterned into left-handed and right-handed, as shown in the inset of Figure 3g. The fabricated left-handed chiral metamaterials exhibit strong absorption of LCP and reflect most RCP at the resonance peak of 1,340 nm. Conversely, the right-handed chiral metamaterials display the opposite behavior, which is consistent with the electromagnetic simulations. The distinct absorption and reflection of CPL lead to a CD greater than 0.7 at 1,340 nm for these chiral metamaterials, as shown in Figure 3g. The complementary intensity in the images of the Vanderbilt University logo, which comprises alternating left-handed and right-handed chiral metamaterials illuminated by L-CPL and R-CPL, further demonstrate the chiroptical properties of the designed patterns (upper panels in Figure 3h). The good contrast of the photocurrent mappings triggered by L-CPL and R-CPL corroborates the CPL detection ability of the fabricated device, which is shown in the lower panels in Figure 3h. In 2022, Namgung et al. synthesized chiral gold (Au) nanoparticles and deposited them onto an achiral IGZO semiconducting channel for a chiral phototransistor[101]. The device structure and measurement conditions are illustrated in the inset of Figure 3i. Due to the different generation rates of hot electrons in these chiral nanoparticles when illuminated by L-CPL and R-CPL, the photocurrent in the fabricated CPL photodetector is influenced by both the tunneling length and light chirality (Figure 3i). This dependence demonstrates the significant potential of this device to distinguish between the polarization state of CPL.
Although various CPL photodetectors have been reported, it must be acknowledged that the development of these devices is still in its infancy. The overwhelming majority of these reports focus on single-device levels, with only a few demonstrating very small-scale device arrays. Significant efforts are needed to explore effective material preparation methods for large-scale chiral thin films and to optimize device architectures for large-scale device arrays.
4. Potential Applications for CPL Photodetectors
Leveraging the unique ability to distinguish between L-CPL and R-CPL, CPL photodetectors exhibit extensive application prospects that go beyond the capabilities of traditional photodetectors. For example, as shown in Figure 4a,b, CPL photodetectors can be utilized as optoelectronic artificial synapses to build a neuromorphic computing system for recognizing images containing information about circular polarization states[25,44,101]. Based on the CPL photodetector, which was enabled by chiral Au nanoparticles and exhibited polarization state-dependent volatile optoelectronic memory behaviors, Namgung et al. simulated an opto-neuromorphic computing system capable of performing image classification work[101]. The simulated computing system comprises an artificial neural network, which consists of 784 input neurons, 300 hidden neurons, and 10 output neurons, and uses the fabricated CPL photodetectors to receive CPL of different wavelengths emitted by the images being recognized. Due to the different light-matter interactions between the chiral Au nanoparticles and the 635-nm and 780-nm lasers, the fabricated CPL photodetector exhibits different volatile current evolutions at these wavelengths. At λ = 635 nm, the fabricated device exhibits a higher post synaptic current (PSC) triggered by R-CPL, and the simulated computing system achieves a higher classification accuracy on the Modified National Institute of Standards and Technology (MNIST) dataset of handwritten digits. Conversely, at λ = 780 nm, L-CPL triggers a higher PSC in the photodetector and results in higher classification accuracy in the computing system. In 2023, Liu et al. reported on an optoelectronic artificial synapse, enabled by a CPL photodetector operating in the UV range[25]. The photoactive material of this CPL photodetector consists of a combination of helical chiral perovskite hybrid and single-wall carbon nanotubes. The circular polarization-dependent photoresponse is attributed to the hole transfer from the perovskite to single-wall carbon nanotubes. In this work, the fabricated CPL photodetector functions as spiking neurons within a simulated spiking neural network designed for classifying the MNIST dataset, which has been pre-converted into a time-varying sequence of spikes. The simulated computing system demonstrates recognition accuracy that is sensitive to the circular polarization state. After training with 300 epochs, each containing a batch of 200 images, the system achieves a recognition accuracy of approximately 0.93 under L-CPL modulation and approximately 0.88 under R-CPL modulation.
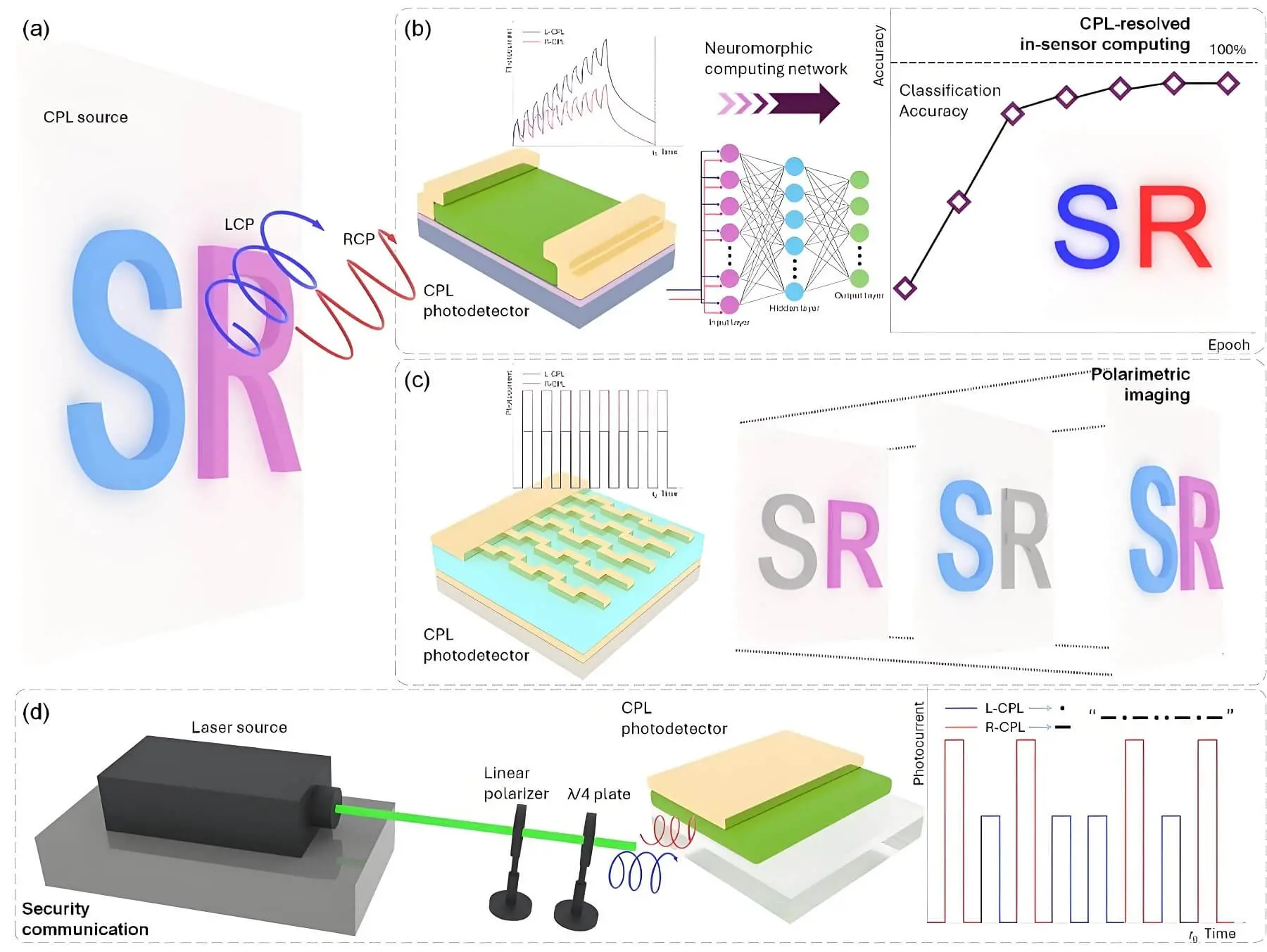
Figure 4. Potential applications of CPL photodetectors. (a) Schematic illustration of a pattern that reflects or emits L-CPL and R-CPL; (b) CPL photodetector used as the sensor in a neuromorphic computing system, enabling CPL-resolved in-sensor computing; (c) CPL photodetector used for polarimetric imaging; (d) schematic illustration of using CPL photodetectors for security communications. CPL: circularly polarized light.
In addition to the potential applications in in-sensor computing systems, CPL photodetectors also hold the promise to be applied in polarimetric imaging[30,52,104]. In 2022, Zhang et al. reported on an optically programmable CPL photodetector using a bilayer semiconductor composed of poly(9,9-di-n-hexylfluorene-alt-benzothiadiazole) (F6BT) and poly(3-hexylthiophene)[52]. Before programming by CPL, the F6BT thin film exhibited no discrimination capability to left-handed and right-handed circular polarization states. However, exposure to L-CPL induced an M-twisted configuration in the F6BT thin films, while R-CPL led to a P-twisted configuration. These changes endowed the photoactive materials with chiroptical properties, achieving a maximum responsivity of 2.76 A/W and an anisotropy factor of 0.425. Leveraging the unique chirality-sensitive photoresponse, the authors further demonstrated a 3 × 3 device array for polarimetric imaging by arranging R-CPL programmed photodetectors along the diagonal and L-CPL programmed photodetectors in the remaining positions. Under illuminations of L-CPL and R-CPL, the array exhibited distinct imaging patterns, that fully demonstrates its application potential for polarimetric imaging. More recently, Wu et al. fabricated a CPL phototransistor by blending the chiral molecule (4-[[4-(hexyloxy)benzoyl]oxy]-1,1'-[1,1'-binaphthyl]-2,2'-diester) benzoic acid (S6N) and poly(9,9-dioctylfluorene)[104]. The chiral transfer induced by annealing between the chiral molecules and the polymers endows this device with a high chiroptical response, achieving a considerable gph = 0.58 at 405-nm CPL. The authors also demonstrated a 3 × 3 device array for polarimetric imaging, which was masked with the letters "H", "F", "U", and "T", respectively, and then irradiated by CPL with different polarization states. Since channel conductivity can be tuned by the gate voltage, they selected Vgs = -80 V and Vds = -80 V to maximize the display contrast. Under the optimized condition, the fabricated device array successfully imaged the "HFUT" patterns.
In traditional optical communication systems, photodetectors detect only light intensity variations without discriminating between the polarization states. In contrast, CPL photodetectors can encode information based on the handedness of CPL light, thereby enabling enhanced security in communication[105,106]. For example, in 2023, Wang et al. fabricated a CPL photodiode by integrating the chiral monomer R5011 into an organic photodetector based on the achiral nematic liquid crystal[106]. Before the formation of a regular helical superstructure, the organic photodetector achieved a high EQE of 70% across the wavelength range of 800 to 870 nm. Additionally, at 870 nm, it reached a peak responsivity of 0.501 A/W and a shot noise-limited specific detectivity of 1.33 × 1014 Jones. During the measurements, the external bias was kept at 0.1 V. After doping chiral compounds into the detection system, the authors reported a high gR of 1.56, along with an impressive D* of 7.12 × 1012 Jones. More importantly, by leveraging its ability to discriminate between L-CPL and R-CPL, the fabricated device can be utilized for encrypted communication. Under illumination of 870-nm L-CPL and R-CPL with the same intensity, the device exhibited stable and distinct photoresponses, generating a significantly higher photocurrent in response to L-CPL than to R-CPL. By interpreting IR-CPL and IL-CPL into short dot signals and long dash signals, respectively, in Morse code, the successful transmission of encrypted information designed as 'NCNST' has been demonstrated. Wang et al. also reported a CPL photodetector with an even higher gR of 1.62[105]. This performance was achieved by incorporating a cholesteric liquid crystal film, chosen for its impressive chiroptical properties, into organic photodiodes. It is worth mentioning that the "inverted quasi-planar heterojunction" structure was employed to further reduce the dark current density, thereby enhancing the photosensitivity. Benefiting from these strategies, the reported device achieved a super-high D* of 1.03 × 1015 Jones at 860 nm. Interestingly, the detection center can be shifted from 530 nm (green) to 640 nm (red) after being exposed to UV light for 20 seconds. Leveraging these fascinating dynamics, the researchers continued to extend the single CPL photodetector to a 1 × 4 CPL photodetector array for double-encrypted communication. Specifically, the initial encryption level utilized the ability of the device to detect CPL for encrypting data via L-CPL and R-CPL conversions, and a secondary encryption involved switching the detection band from green to red under specific conditions. This adjustment of certain pixels in the array, such as shifting the third pixel in the array from green to red, ensured encrypted transmission. Only devices configured with specific transformations could decode the true data, while traditional CPL detectors received distorted information. Table 1 summarizes the potential applications and requirements for CPL photodetectors, emphasizing the universal demand for devices with strong discrimination capabilities between L-CPL and R-CPL. As highlighted in this review, material innovation that enhances chiroptical properties is crucial. Future high-performance CPL photodetectors are anticipated to provide significant advantages, including a smaller device footprint, higher energy efficiency, and enhanced discrimination capabilities, compared with conventional CPL detection strategies.
Potential applications | Requirements for CPL photodetectors |
Neuromorphic computing | Strong discrimination capability between L-CPL and R-CPL and optoelectronic memory conductive states triggered by CPL |
Polarimetric imaging | Strong discrimination capability, fast response speed, and high sensitivity, large photocurrent/dark current ratio to CPL |
Security communications | Strong discrimination capability, fast response speed, and large signal to noise ratio to CPL |
CPL: circularly polarized light.
The applications mentioned above represent only a small fraction of the potential uses for CPL photodetectors. There remains a vast realm of possibilities for researchers to explore and utilize CPL photodetectors to revolutionize our daily lives.
5. Conclusions and Outlooks
CPL photodetectors, capable of discerning the circular polarization states of light, offer a new playground for humans to play with light. The past decades have witnessed significant advances in research on CPL photodetectors, resulting in many important achievements. In this review, we first introduce common chiral materials used for CPL detection, which primarily consist of chiral organic materials, chiral organic-inorganic hybrid perovskites, and other chiral compounds. Among these, chiral organic materials can be further categorized into chiral molecules, chiral supramolecular structures, and chiral conjugated polymers. Additionally, other chiral materials, including chiral COFs, chiral MOFs, and chiral metal nanoclusters, have also been reported for CPL detection. Then, we summarize the figures of merit used to evaluate the performance of CPL photodetectors. One of the most critical parameters is the dissymmetry factor (gph or gres), which tells how effectively a CPL photodetector can discriminate between L-CPL and R-CPL. In addition to the dissymmetry factor, other performance metrics including responsivity, EQE, D*, and response time should also be assessed under illumination of L-CPL and R-CPL, respectively. This comprehensive evaluation helps fully characterize the performance of CPL photodetectors. After that, we introduce representative works about CPL photodetectors, categorizing them based on their architecture into lateral structures, vertical structures, and those enabled by chiral metamaterials. Next, we depict application prospects of CPL photodetectors and highlight their applications in CPL-resolved in-sensor computing, polarimetric imaging, and secure communication. In the end, we offer an outlook on the challenges encountered in the research field of CPL photodetectors.
Although significant progress has been made in the development of CPL photodetectors, they are still far away from practical implementation, and many unresolved issues require satisfactory solutions. 1) The discrimination capability of most reported CPL photodetectors is limited to distinguishing between the well-defined L-CPL and R-CPL with the same intensity. At the current stage, the most direct evidence supporting the ability of a reported CPL photodetector to discriminate between L-CPL and R-CPL lies in their different photocurrent responses to L-CPL and R-CPL of the same intensity. In laboratories, these two states must be predefined using additional optical components, including a combination of a linear polarizer and a λ/4 plate, and the light power of these two polarization states needs to remain the same, as verified by a commercial laser power meter. In other words, the CPL photodetector cannot determine the circular polarization state of a CPL with unknown intensity, much less under conditions of non-ideal CPL. Given that the polarization states and intensity of light typically vary after transmission through a dielectric in real world, the stringent operating requirements severely limit the practical applications of CPL photodetectors. 2) The chiroptical properties of most reported chiral materials used in CPL photodetectors are derived from chiral organic components, which often suffer from poor thermal stability and small carrier mobilities. The limited thermal budget of these chiral materials impedes the integration of CPL photodetectors based on these materials with monolithic complementary-metal-oxide-semiconductor circuits for commercial products. In addition, due to the very small carrier mobilities in organic semiconductors, CPL photodetectors based on these chiral materials usually present a slow response speed. This deficiency is not conducive to their applications in optical communications, where a high response speed is required for a large communication bandwidth. On the other hand, integrating chiral metamaterials with semiconductors that have large carrier mobilities could help mitigate this issue. However, the limited injection efficiency of hot carriers generated under illumination into semiconducting channels may deteriorate the dissymmetry factors. 3) The design and fabrication of CPL photodetectors remain at a single-device level. Although small-scale CPL photodetector arrays (e.g., 3 × 3) have been demonstrated, their pixel resolution is too low for practical polarimetric imaging applications. In addition, the feature sizes of reported single devices typically range from hundreds of micrometers to even millimeters. Such large device dimensions hamper subsequent system miniaturization and result in high energy consumption.
Given these looming challenges, the following research directions could be pursued in this research field (Figure 5): 1) Optimize CPL photodetector design to directly determine circular polarization states of CPLs with unknown intensities. A possible design approach is to place two CPL photodetectors made from a pair of enantiomers in parallel, as illustrated in Figure 5a. By leveraging the distinct photoresponses of these enantiomers to L-CPL or R-CPL, both the polarization state and intensity of the incident CPLs can be directly determined through the readout photocurrents in these two CPL photodetectors. For example, consider the scenario where both the L-Device and R-Device are illuminated by a CPL with an unknown circular polarization state and unknown intensity. After measuring the photocurrent Iph,1 from the L-Device, we can infer that the power of the incident CPL could be either P1 or P2 (Figure 5b). Meanwhile, by reading the photocurrent Iph,2 from the R-Device, the other two possible CPL power values, P1' and P3, can be determined (Figure 5c). Given that P1 ≈ P1' and P2 > P3, we can conclude that the circular polarization state of the incident CPL is left-handed, and the intensity is P1. 2) Explore inorganic materials with chiroptical properties for CPL photodetection. Compared to organic materials, inorganic materials usually present higher thermal stability and larger carrier mobilities. For instance, based on the moiré-induced strong symmetry breaking and quantum geometric contribution in twisted double layer graphene (TDBG), Ma et al. reported the tunable bulk photovoltaic effect (BPVE) in TDBG (Figure 5d)[107]. The light polarization state-dependent photoresponse can be tuned using external electric fields for intelligent imaging applications. Leveraging the interactions between CPL and other emerging 2D materials such as black phosphorous, transition metal dichalcogenides, and van der Waals (vdW) heterostructures, there is significant potential to fabricate high-performance CPL photodetectors from this inorganic material platform[108]. It is worth specifically mentioning that tellurium (Te), selenium (Se), and their alloy nanocrystals, another fascinating group of vdW materials with many intriguing properties, exhibit an intrinsic chiral crystal structure[109-113]. This unique structure is clearly identified in their transmission electronic microscopic images, and the circular photogalvanic and photovoltaic effects have also been discovered in 2D Te[114-120]. Then how to develop high-performance CPL photodetectors based on these materials could be an intriguing research topic in the future. Besides vdW materials, other twisted inorganic films, for example, chiral moiré stacking films made from zinc oxide nanowires, can also be used to fabricate CPL photodetectors[121]. The high thermal stability, large-scale growth, and considerable carrier mobilities of these materials holds the great promise for advancing the integration of high-performance CPL photodetectors with processing chips. 3) Develop preparation strategies to produce high-quality thin films with superior chiroptical properties for demonstrating a large-scale CPL detector array with high device uniformity. The successful demonstration of a large-scale photodetector array is essential to promote the step of CPL photodetectors into industrial applications, which requires the preparation of high-quality chiral thin films on a large scale (Figure 5e). Current methods, which primarily involve drop-casting and spin-coating chiral organic materials dissolved into organic solutions, often result in uneven thickness and significant device-to-device variation. Instead, new preparation methods such as fast deposition method and orientation filter funnel method for high-quality organic thin films could be investigated and applied into the preparation of large-scale and high-quality chiral thin films[122-124]. In addition to these promising research directions in CPL detection system design and fabrication, substantial efforts should also be directed towards investigating the potential application prospects of CPL photodetection. For instance, many active molecules in living organisms exhibit intrinsic chirality, potentially leading to unique interactions with CPL. Consequently, CPL photodetectors could find applications in fields such as bioimaging, biosensing, and even drug screening.
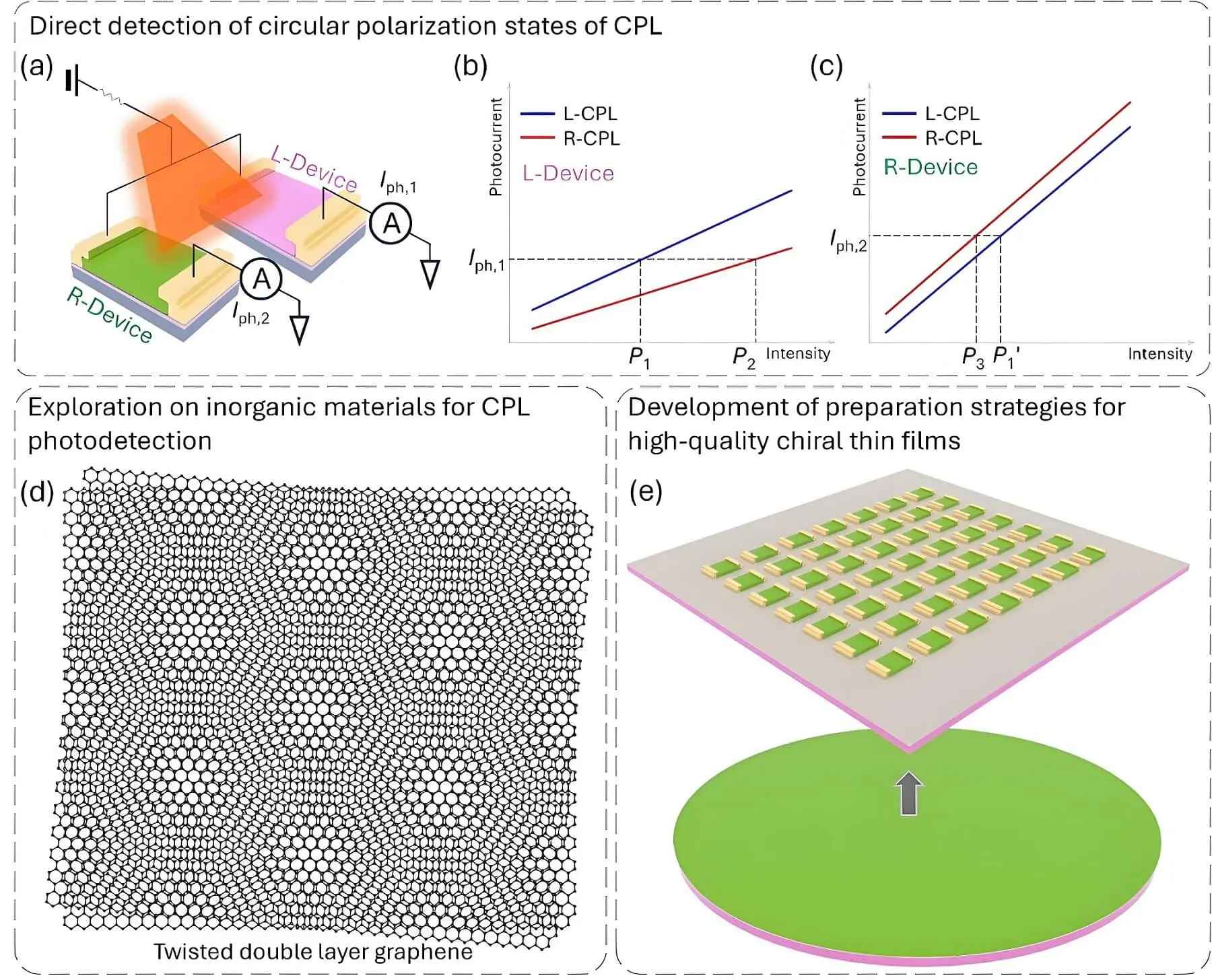
Figure 5. Schematic illustrations of potential research directions. Direct detection of CPLs with unknown circular polarization states and intensities: (a) schematic illustration of the detection system; intensity-dependent photocurrent in (b) L-Device and (c) R-Device; (d) exploration on novel material platform for CPL photodetection; (e) development of preparation strategies of high-quality chiral thin films in large-scale and subsequent design and fabrication of large CPL photodetector arrays. CPL: circularly polarized light.
Authors contribution
Zha J, Tan C: Data analysis and interpretation, article conception and design, writing, review, editing.
Huang H: Writing, review, editing.
Zhang Q: Article conception and design, writing, review, editing.
All authors approved the final version of the manuscript.
Conflicts of interest
Chaoliang Tan is an Associate Editor of Smart Materials and Devices. Other authors declared that there are no conflicts of interest.
Ethical approval
Not applicable.
Consent to participate
Not applicable.
Consent for publication
Not applicable.
Availability of data and materials
Not applicable.
Funding
C.T. thanks the funding support from the National Natural Science Foundation of China - Excellent Young Scientists Fund (Hong Kong and Macau) (52122002), the Start-Up Grant (Project No. 9610710) from City University of Hong Kong, the ECS scheme (21201821) from the Research Grant Council of Hong Kong and ITC via Hong Kong Branch of National Precious Metals Material Engineering Research Center (NPMM).
Copyright
© The Author(s) 2025.
References
-
1. Zubairy M. A very brief history of light. In: Al-Amri MD, El-Gomati M, Zubairy M, editors. Optics in Our Time. Switzerland: Springer; 2016. p. 3-24.
[DOI] -
2. Zeilinger A. Experiment and the foundations of quantum physics. Rev Mod Phys. 1999;71(2):S288-S297.
[DOI] -
3. Richardson FS, Riehl JP. Circularly polarized luminescence spectroscopy. Chem Rev. 1977;77(6):773-792.
[DOI] -
4. Ruggenthaler M, Tancogne-Dejean N, Flick J, Appel H, Rubio A. From a quantum-electrodynamical light-matter description to novel spectroscopies. Nat Rev Chem. 2018;2:0118.
[DOI] -
5. Farshchi R, Ramsteiner M, Herfort J, Tahraoui A, Grahn HT. Optical communication of spin information between light emitting diodes. Appl Phys Lett. 2011;98:162508.
[DOI] -
6. Lodahl P, Mahmoodian S, Stobbe S, Rauschenbeutel A, Schneeweiss P, Voltz J, et al. Chiral quantum optics. Nature. 2017;541:473-480.
[DOI] -
7. Zhang MJ, Guo Q, Li Z, Zhou Y, Zhao S, Tong Z, et al. Processable circularly polarized luminescence material enables flexible stereoscopic 3D imaging. Sci Adv. 2023;9(43):eadi9944.
[DOI] -
8. Kunnen B, Macdonald C, Doronin A, Jacques S, Eccles M, Meglinski I. Application of circularly polarized light for non-invasive diagnosis of cancerous tissues and turbid tissue-like scattering media. J Biophotonics. 2015;8(4):317-323.
[DOI] -
9. Chen Y, Yang X, Gao J. 3D Janus plasmonic helical nanoapertures for polarization-encrypted data storage. Light Sci Appl. 2019;8:45.
[DOI] -
10. Miles AJ, Janes RW, Wallace BA. Tools and methods for circular dichroism spectroscopy of proteins: a tutorial review. Chem Soc Rev. 2021;50:8400-8413.
[DOI] -
11. Kaminsky W, Claborn K, Kahr B. Polarimetric imaging of crystals. Chem Soc Rev. 2004;33(8):514-525.
[DOI] -
12. Liu Y, Xing P. Circularly polarized light responsive materials: design strategies and applications. Adv Mater. 2023;35(49):2300968.
[DOI] -
13. Gilot J, Abbel R, Lakhwani G, Meijer EW, Schenning APHJ, Meskers SCJ. Polymer photovoltaic cells sensitive to the circular polarization of light. Adv Mater. 2010;22(20):E131-E134.
[DOI] -
14. Yang Y, da Costa RC, Fuchter MJ, Campbell AJ. Circularly polarized light detection by a chiral organic semiconductor transistor. Nature Photon. 2013;7:634-648.
[DOI] -
15. Mori K, Murase T, Fujita M. One-step synthesis of [16]helicene. Angew Chem Int Ed Engl. 2015;54(23):6847-6851.
[DOI] -
16. Schulz M, Balzer F, Scheunemann D, Arteaga O, Lützen A, Meskers S, et al. Chiral excitonic organic photodiodes for direct detection of circular polarized light. Adv Funct Mater. 2019;29(16):1900684.
[DOI] -
17. Wang Z, Gao M, Hao X, Qin W. Helical-chiroptical nanowires generated orbital angular momentum for the detection of circularly polarized light. Appl Phys Lett. 2020;116:053301.
[DOI] -
18. Qiu Z, Ju CW, Frédéric L, Hu Y, Schollmeyer D, Pieters G, et al. Amplification of dissymmetry factors in π-extended [7]- and [9]helicenes. J Am Chem Soc. 2021;143(12):4661-4667.
[DOI] -
19. Zhang L, Song I, Ahn J, Han M, Linares M, Surin M, et al. π-Extended perylene diimide double-heterohelicenes as ambipolar organic semiconductors for broadband circularly polarized light detection. Nat Commun. 2021;12:142.
[DOI] -
20. Ward MD, Wade J, Shi X, Nelson J, Campbell AJ, Fuchter MJ. Highly selective high-speed circularly polarized photodiodes based on π-conjugated polymers. Adv Optical Mater. 2022;10(2):2101044.
[DOI] -
21. Chen C, Gao L, Gao W, Ge C, Du X, Li Z, et al. Circularly polarized light detection using chiral hybrid perovskite. Nat Commun. 2019;10:1927.
[DOI] -
22. Ma J, Fang C, Chen C, Jin L, Wang J, Wang S, et al. Chiral 2D perovskites with a high degree of circularly polarized photoluminescence. ACS Nano. 2019;13(3):3659-3665.
[DOI] -
23. Ishii A, Miyasaka T. Direct detection of circular polarized light in helical 1D perovskite-based photodiode. Sci Adv. 2020;6:eabd3274.
[DOI] -
24. Zhao Y, Qiu Y, Feng J, Zhao J, Chen G, Gao H, et al. Chiral 2D-perovskite nanowires for stokes photodetectors. J Am Chem Soc. 2021;143(22):8437-8445.
[DOI] -
25. Liu Q, Wei Q, Ren H, Zhou L, Zhou Y, Wang P, et al. Circular polarization-resolved ultraviolet photonic artificial synapse based on chiral perovskite. Nat Commun. 2023;14:7179.
[DOI] -
26. Gu Q, Zha J, Chen C, Wang X, Yao W, Liu J, et al. Constructing chiral covalent-organic frameworks for circularly polarized light detection. Adv Mater. 2024;36(17):2306414.
[DOI] -
27. Tang X, Zha J, Wu X, Tong J, Gu Q, Zhang K, et al. Construction of chiral covalent organic frameworks through a linker decomposition chiral induction strategy for circularly polarized light detection. Angew Chem Int Ed. 2024;64(1):e202413675.
[DOI] -
28. He WM, Zha J, Zhou Z, Cui YJ, Luo P, Ma L, et al. Atomically precise chiral metal nanoclusters for circularly polarized light detection. Angew Chem Int Ed. 2024;63(34):e202407887.
[DOI] -
29. Kuzyk A, Schreiber R, Fan Z, Pardatscher G, Roller E, Högele A, et al. DNA-based self-assembly of chiral plasmonic nanostructures with tailored optical response. Nature. 2012;483:311-314.
[DOI] -
30. Li W, Coppens ZJ, Besteiro LV, Wang W, Govorov AO, Valentine J. Circularly polarized light detection with hot electrons in chiral plasmonic metamaterials. Nat Commun. 2015;6:8379.
[DOI] -
31. Lee SH, Singh DP, Sung JH, Jo MH, Kwon KC, Kim SY, et al. Highly photoresponsive and wavelength-selective circularly-polarized-light detector based on metal-oxides hetero-chiral thin film. Sci Rep. 2016;6:19580.
[DOI] -
32. Grey P, Fernandes SN, Gaspar D, Fortunato E, Martins R, Godinho M, et al. Field-effect transistors on photoinc cellulose nanocrystal solid electrolyte for circular polarized light sensing. Adv Funct Mater. 2019;29(21):1805279.
[DOI] -
33. Grey P, Chapa M, Alexandre M, Materus T, Fortunato E, Martins R, et al. Combining soft with hard condensed matter for circular polarized light sensing and logic operations. Adv Optical Mater. 2021;9(6):2001731.
[DOI] -
34. Shang X, Wan L, Wang L, Gao F, Li H. Emerging materials for circularly polarized light detection. J Mater Chem C. 2022;10:2400-2410.
[DOI] -
35. Zhang C, Wang X, Qiu L. Circularly polarized photodetectors based on chiral materials: a review. Front Chem. 2021;9:711488.
[DOI] -
36. Shi W, Salerno F, Ward MD, Santana-Bonilla A, Wade J, Hou X, et al. Fullerene desymmetrization as a means to achieve single-enantiomer electron acceptors with maximized chiroptical responsiveness. Adv Mater. 2021;33(1):2004115.
[DOI] -
37. Shang X, Song I, Ohtsu H, Lee Y, Zhao T, Kojima T, et al. Supramolecular nanostructures of chiral perylene diimides with amplified chirality for high-performance chiroptical sensing. Adv Mater. 2017;29(21):1605828.
[DOI] -
38. Hao J, Lu H, Mao L, Chen X, Beard M, Blackburn JL. Direct detection of circularly polarized light using chiral copper chloride-carbon nanotube heterostructures. ACS Nano. 2021;15(4):7608-7617.
[DOI] -
39. Shang X, Song I, Lee JH, Choi W, Ahn J, Ohtsu H, et al. Surface-doped quasi-2D chiral organic single crystals for chiroptical sensing. ACS Nano. 2020;14(10):14146-14156.
[DOI] -
40. Li D, Liu X, Wu W, Peng Y, Zhao S, Li L, et al. Chiral lead-free hybrid perovskites for self-powered circularly polarized light detection. Angew Chem Int Ed. 2021;133(15):8415-8418.
[DOI] -
41. Han H, Lee YJ, Kyhm J, Jeong JS, Han JH, Yang MY, et al. High-performance circularly polarized light-sensing near-infrared organic phototransistors for optoelectronic cryptographic primitives. Adv Funct Mater. 2020;30(52):2006236.
[DOI] -
42. Wang L, Xue Y, Cui M, Huang Y, Xu H, Qin C, et al. A chiral reduced-dimension perovskite for an efficient flexible circularly polarized light photodetector. Angew Chem Int Ed. 2020;132(16):6442-6450.
[DOI] -
43. Han H, Choi JH, Ahn J, Lee H, Choi C, Jung W, et al. Chiral diketopyrrolopyrrole-based conjugated polymers with intramolecular rotation-isomeric conformation asymmetry for near-infrared circularly polarized light-sensing organic phototransistors. ACS Appl Mater Inter. 2023;15(49):57447-57460.
[DOI] -
44. Lee H, Hwang JH, Song SH, Han H, Han SJ, Suh BL, et al. Chiroptical synaptic heterojunction phototransistors based on self-assembled nanohelix of π-conjugated molecules for direct noise-reduced detection of circularly polarized light. Adv Sci. 2023;10(27):2304039.
[DOI] -
45. Choi JH, Hwang HS, Jang HB, Kim SU, Park HL. Flexible phototransistors integrated with chiral liquid crystal encapsulating film for improving color selectivity and stability. ACS Appl Electron Mater. 2024;6(11):8094-8103.
[DOI] -
46. Peng Y, Liu X, Li L, Yao Y, Ye H, Shang X, et al. Realization of vis-NIR dual-modal circularly polarized light detection in chiral perovskite bulk crystals. J Am Chem Soc. 2021;143(35):14077-14082.
[DOI] -
47. Wu W, Li L, Li D, Yao Y, Xu Z, Liu X, et al. Tailoring the distinctive chiral-polar perovskites with alternating cations in the interlayer space for self-driven circularly polarized light detection. Adv Optical Mater. 2022;10(18):2102678.
[DOI] -
48. Li X, Wu F, Yao Y, Wu W, Ji C, Li L, et al. Robust spin-dependent anisotropy of circularly polarized light detection from achiral layered hybrid perovskite ferroelectric crystals. J Am Chem Soc. 2022;144(31):14031-14036.
[DOI] -
49. Liu Z, Zhang C, Liu X, Ren A, Zhou Z, Qiao C, et al. Chiral hybrid perovskite single-crystal nanowire arrays for high-performance circularly polarized light detection. Adv Sci. 2021;8(21):2102065.
[DOI] -
50. Kim NY, Kyhm J, Han H, Kim J, Ahn SJ, Hwang DK, et al. Chiroptical-conjugated polymer/chiral small molecule hybrid thin films for circularly polarized light-detecting heterojunction devices. Adv Funct Mater. 2019;29(11):1808668.
[DOI] -
51. Liu L, Wei Z, Meskers SCJ. Semi-transparent, chiral organic photodiodes with incident direction-dependent selectivity for circularly polarized light. Adv Mater. 2023;35(10):2209730.
[DOI] -
52. Zhang C, Xu C, Chen C, Cheng J, Zhang H, Ni F, et al. Optically programmable circularly polarized photodetector. ACS Nano. 2022;16(8):12452-12461.
[DOI] -
53. Yao B, Wei Q, Yang Y, Zhou W, Jiang X, Wang H, et al. Symmetry-broken 2D lead–tin mixed chiral perovskite for high asymmetry factor circularly polarized light detection. Nano Lett. 2023;23(5):1938-1945.
[DOI] -
54. Xiao W, Shi X, Zhang Y, Peng W, Zeng Y. Circularly polarized light detector based on 2D embedded chiral nanostructures. Phys Scr. 2019;94(8):085501.
[DOI] -
55. Shi X, Xiao W, Fan Q, Zhou T, Song W, Zhang C, et al. Circularly polarized light photodetector based on X-shaped chiral metamaterial. IEEE Sens J. 2018;18(22):9203-9206.
[DOI] -
56. Hu Y, Wang Y, Sang T, Yang G. Mid-infrared circular-polarization-sensitive photodetector based on a chiral metasurface with a photothermoelectric effect. Appl Opt. 2023;62(9):2292-2299.
[DOI] -
57. Zhang G, Lyu X, Qin Y, Li Y, Fan Z, Meng X, et al. High discrimination ratio, broadband circularly polarized light photodetector using dielectric achiral nanostructures. Light Sci Appl. 2024;13:275.
[DOI] -
58. Rajamani S, Simeone D, Pecora A, Manoccio M, Balestra G, Bayramov A, et al. Circularly polarized light detection through 3D chiral metasurface-based phototransistors. Adv Mater Technol. 2024;9(3):2301250.
[DOI] -
59. Hong J, van de, Lee N, Kim SJ, Lalanne P, Kik PG. Nonlocal metasurface for circularly polarized light detection. Optica. 2023;10(1):134-141.
[DOI] -
60. Yang D, Ma D. Development of organic semiconductor photodetectors: from mechanism to applications. Adv Optical Mater. 2019;7(1):1800522.
[DOI] -
61. Chow PCY, Someya T. Organic photodetectors for next-generation wearable electronics. Adv Mater. 2020;32(15):1902045.
[DOI] -
62. Ren H, Chen JD, Li YQ, Tang JX. Recent progress in organic photodetectors and their applications. Adv Sci. 2021;8(1):2002418.
[DOI] -
63. Ahn J, Lee SH, Song I, Chidchob P, Kwon Y, Oh JH. Chiral organic semiconducting materials for next-generation optoelectronic sensors. Device. 2023;1(5):100176.
[DOI] -
64. Zhu D, Jiang W, Ma Z, Feng J, Zhan X, Lu C, et al. Organic donor-acceptor heterojunctions for high performance circularly polarized light detection. Nat Commun. 2022;13:3454.
[DOI] -
65. Ding X, Wei C, Wang L, Yang J, Huang W, Chang Y, et al. Multicomponent flexible organic crystals. SmartMat. 2024;5(4):e1213.
[DOI] -
66. Cheng Y, Yang SH, Hsu CS. Synthesis of conjugated polymers for organic solar cell applications. Chem Rev. 2009;109(11):5868-5923.
[DOI] -
67. Kertesz M, Choi HC, Yang S. Conjugated polymers and aromaticity. Chem Rev. 2005;105(10):3448-3481.
[DOI] -
68. Shen Y, Chen CF. Helicenes: synthesis and applications. Chem Rev. 2012;112(3):1463-1535.
[DOI] -
69. Takenaka N, Sarangthem RS, Captain B. Helical chiral pyridine n-oxides: a new family of asymmetric catalysts. Angew Chem Int Ed. 2008;47(50):9708-9710.
[DOI] -
70. Yang Y, Liu L, Wei Z. Chiral conjugated molecular assemblies interact with substances and light. Acc Mater Res. 2024;5(3):329-346.
[DOI] -
71. Kwon Y, Jung J, Lee WB, Oh J. Axially chiral organic semiconductors for visible-blind UV-selective circularly polarized light detection. Adv Sci. 2024;11(14):2308262.
[DOI] -
72. Wang H, Yao L, Zhan Y, Yu H, Wu S, Liu X. Self-powered circularly polarized light photodetector with high responsivity based on the chiral quasi-2D perovskite film. ACS Appl Mater Inter. 2025;17(2):3716-3724.
[DOI] -
73. Zhang X, Xu Y, Alphenaar AN, Ramakrishnan S, Zhang Y, Babatunde A, et al. Self-powered circularly polarized light detection enabled by chiral two-dimensional perovskite with mixed chiral-achiral organic cations. ACS Nano. 2024;18(22):14605-14616.
[DOI] -
74. Thilgen C, Diederich F. Structural aspects of fullerene chemistry-a journey through fullerene chirality. Chem Rev. 2006;106(12):5049-5135.
[DOI] -
75. Lenes M, Wetzelaer GAH, Kooistra FB, Veenstra SC, Hummelen JC, Blom PWM. Fullerene bisadducts for enhanced open-circuit voltages and efficiencies in polymer solar cells. Adv Mater. 2008;20(11):2116-2119.
[DOI] -
76. Lenes M, Shelton SW, Sieval AB, Kronholm DF, Hummelen JC, Blom P. WM Electron trapping in higher adduct fullerene-based solar cells. Adv Funct Mater. 2009:19(18):3002-3007;
[DOI] -
77. Liu M, Zhang L, Wang T. Supramolecular chirality in self-assembled systems. Chem Rev. 2015;115(15):7304-7397.
[DOI] -
78. Liu L, Yang Y, Meskers SCJ, Wang Q, Zhang L, Yang C, et al. Fused-ring electron-acceptor single crystals with chiral 2D supramolecular organization for anisotropic chiral optoelectronic devices. Adv Mater. 2023;35(45):2304627.
[DOI] -
79. Shang X, Song I, Han M, Lee JH, Ohtsu H, Choi W, et al. "Majority-rules" effect on supramolecular chirality and optoelectronic properties of chiral tetrachloro-perylene diimides. Adv Optical Mater. 2021;9(6):2001911.
[DOI] -
80. Liu L, Yang Y, Wang Y, Adil MA, Zhao Y, Zhang J, et al. Building supramolecular chirality in bulk heterojunctions enables amplified dissymmetry current for high-performing circularly polarized light detection. ACS Mater Lett. 2022;4(2):401-409.
[DOI] -
81. Liu L, Yang Y, Zhu L, Zhang J, Chen K, Wei Z. Chiral non-fullerene acceptor enriched bulk heterojunctions enable high-performance near-infrared circularly polarized light detection. Small. 2022;18(31):2202941.
[DOI] -
82. Wang J, Fang C, Ma J, Wang S, Jin L, Li W, Li D. Aqueous synthesis of low-dimensional lead halide perovskites for room-temperature circularly polarized light emission and detection. ACS Nano. 2019;13(8):9473-9481.
[DOI] -
83. Wang J, Lu H, Pan X, Xu J, Liu H, Liu X, et al. Spin-dependent photovoltaic and photogalvanic responses of optoelectronic devices based on chiral two-dimensional hybrid organic-inorganic perovskites. ACS Nano. 2021;15(1):588-595.
[DOI] -
84. Liu T, Shi W, Tang W, Liu Z, Schroeder BC, Fenwick O, et al. High responsivity circular polarized light detectors based on quasi two-dimensional chiral perovskite films. ACS Nano. 2022;16(2):2682-2689.
[DOI] -
85. Wu W, Shang X, Xu Z, Ye H, Yao Y, Chen X, et al. Toward efficient two-photon circularly polarized light detection through cooperative strategies in chiral quasi-2D perovskites. Adv Sci. 2023;10(9):2206070.
[DOI] -
86. Zhuang B, Jin Z, Tian D, Zhu S, Zeng L, Fan J, et al. Halogen regulation for enhanced luminescence in emerging (4-HBA)SbX5·H2O perovskite-like single crystals. Acta Phys Chim Sin. 2023;39(1):2209007.
[DOI] -
87. Peña MA, Fierro JLG. Chemical structures and performance of perovskite oxides. Chem Rev. 2001;101(7):1981-2018.
[DOI] -
88. Hong X, Liu X, Liao L, Zou X. Review on metal halide perovskite-based optoelectronic synapses. Photon Res. 2023;11(5):787-807.
[DOI] -
89. Ferreira R, Shaikh M, Jakka SK, Deuermeier J, Barquinha P, Ghosh S, et al. Bandlike transport in FaPbBr3 quantum dot phototransistor with high hole mobility and ultrahigh photodetectivity. Nano Leet. 2022;22(22):9020-9026.
[DOI] -
90. Mustaqeem M, Kamal S, Ahmad N, Chou PT, Lin KH, Huang YC, et al. Chiral metal-organic framework based spin-polarized flexible photodetector with ultrahigh sensitivity. Mater Today Nano. 2023;21:100303.
[DOI] -
91. Wang X, Zhang Q. Organic quantum materials: A review. SmartMat. 2023;4(4):e1196.
[DOI] -
92. Yuan G, Xi Z, Wang C, Sun X, Han J, Guo R. Construction of supramolecular chiral polyaniline-gold nanocomposite as nanozyme for enantioselective catalysis. Acta Phys Chim Sin. 2023;39(7):2212061.
[DOI] -
93. Zha J, Luo M, Ye M, Ahmed T, Yu X, Lien DH, et al. Infrared photodetectors based on 2D materials and nanophotonics. Adv Funct Mater. 2022;32(15):2111970.
[DOI] -
94. Hu R, Qin W. Review of polarized light-spin/dipole interactions: fundamental physics and application in circularly polarized detecting. Laser Photonics Rev. 2024;19(3):2400761.
[DOI] -
95. Song I, Ahn J, Ahn H, Lee SH, Mei J, Kotov NA, et al. Helical polymers for dissymmetric circularly polarized light imaging. Nature. 2023;617:92-99.
[DOI] -
96. Wan L, Zhang R, Cho E, Li H, Coropceanu V, Brédas JL, et al. Sensitive near-infrared circularly polarized light detection via non-fullerene acceptor blends. Nat Photonics. 2023;17:649-655.
[DOI] -
97. Baeg KJ, Binda M, Natali D, Caironi M, Noh YY. Organic light detectors: photodiodes and phototransistors. Adv Mater. 2013;25(31):4267-4295.
[DOI] -
98. Wang C, Zhang X, Hu W. Organic photodiodes and phototransistors toward infrared detection: materials, devices, and applications. Chem Soc Rev. 2020;49(3):653-670.
[DOI] -
99. Dickey KC, Anthony JE, Loo YL. Improving organic thin-film transistor performance through solvent-vapor annealing of solution-processable triethylsilylethynyl anthradithiophene. Adv Mater. 2006;18(13):1721-1726.
[DOI] -
100. Wang C, Li G, Dai Z, Tian W, Li L. Patterned chiral perovskite film for self-driven stokes photodetectors. Adv Funct Mater. 2024;34(25):2316265.
[DOI] -
101. Namgung SD, Kim RM, Lim YC, Lee JW, Cho NH, Kim H, et al. Circularly polarized light-sensitive, hot electron transistor with chiral plasmonic nanoparticles. Nat Commun. 2022;13:5081.
[DOI] -
102. Liu C, Zuo X, Xu S, Wang L, Xiong D. High circular polarization recognition quantum well infrared photodetector based on a chiral metamaterial microcavity. J Opt Soc Am B. 2022;39(6):1520-1527.
[DOI] -
103. Ashalley E, Ma CP, Zhu YS, Xu HX, Yu P, Wang ZM. Recent progress in chiral absorptive metamaterials. J Electron Sci Technol. 2021;19(3):100098.
[DOI] -
104. Wu X, Liu J, Xu Y, Qiu L, Wang X. High-performance circularly polarized photodetectors based on chiral transfer of achiral poly(9,9-dioctylfluorene). J Mater Chem C. 2025;13:628-638.
[DOI] -
105. Wang Q, Bao J, Zhang Y, Wang Y, Qiu D, Yang J, et al. High-performance organic narrow dual-band circular polarized light detection for encrypted communications and color imaging. Adv Mater. 2024;36(16):2312396.
[DOI] -
106. Wang Q, Bao J, Liu L, Yang Y, Yang J, Gao H, et al. High-performance near-infrared narrowband circularly polarized light organic photodetectors. Nano Today. 2024;54:102132.
[DOI] -
107. Ma C, Yuan S, Cheung P, Watanabe K, Taniguichi T, Zhang F, et al. Intelligent infrared sensing enabled by tunable moiré quantum geometry. Nature. 2022;604:266-272.
[DOI] -
108. Rong R, Liu Y, Nie X, Zhang W, Zhang Z, Liu Y, et al. The interaction of 2D materials with circularly polarized light. Adv Sci. 2023;10(10):2206191.
[DOI] -
109. Zha J, Dong D, Huang H, Xia Y, Tong J, Liu H, et al. Electronics and optoelectronics based on tellurium. Adv Mater. 2024;36(45):2408969.
[DOI] -
110. Zha J, Tong J, Huang H, Xia Y, Dong D, Tan C. A perspective on tellurium-based optoelectronics. Appl Phys Lett. 2024;125(7):070504.
[DOI] -
111. Wang Y, Qiu G, Wang R, Huang S, Wang Q, Liu Y, et al. Field-effect transistors made from solution-grown two-dimensional tellurene. Nat Electron. 2018;1:228-236.
[DOI] -
112. Qin J, Qiu G, Jian J, Zhou H, Yang L, Charnas A, et al. Controlled growth of a large-size 2D selenium nanosheet and its electronic and optoelectronic applications. ACS Nano. 2017;11(10):10222-10229.
[DOI] -
113. Huang H, Zha J, Xu S, Yang P, Xia Y, Wang H, et al. Precursor-confined chemical vapor deposition of 2D single-crystalline SexTe1-x nanosheets for p-type transistors and inverters. ACS Nano. 2024;18(26):17293-17303.
[DOI] -
114. Qiu G, Niu C, Wang Y, Si M, Zhang Z, Wu W, et al. Quantum Hall effect of Weyl fermions in n-type semiconducting tellurene. Nat Nanotechnol. 2020;15:585-591.
[DOI] -
115. Qin JK, Liao PY, Si M, Gao S, Qiu G, Jian J, et al. Raman response and transport properties of tellurium atomic chains encapsulated in nanotubes. Nat Electron. 2020;3:141-147.
[DOI] -
116. Ben-Moshe A, Silva A, Müller A, Abu-odeh A, Harrison P, Waelder J, et al. The chain of chirality transfer in tellurium nanocrystals. Science. 2021;372(6543):729-733.
[DOI] -
117. Niu C, Huang S, Ghosh N, Tan P, Wang M, Wu W, et al. Tunable circular photogalvanic and photovoltaic effect in 2D tellurium with different chirality. Nano Lett. 2023;23(8):3599-3606.
[DOI] -
118. Ben-Moshe A, Wolf SG, Sadan MB, Houben L, Fan Z, Govorov AO, et al. Enantioselective control of lattice and shape chirality in inorganic nanostructures using chiral biomolecules. Nat Commun. 2014;5:4302.
[DOI] -
119. Zhao C, Tan C, Lien DH, Song X, Amani M, Hettick M, et al. Evaporated tellurium thin films for p-type field-effect transistors and circuits. Nat Nanotechnol. 2020;15:53-58.
[DOI] -
120. Tan C, Amani M, Zhao C, Hettick M, Song X, Lien D, et al. Evaporated SexTe1-x thin films with tunable bandgaps for short-wave infrared photodetectors. Adv Mater. 2020;32(38):2001329.
[DOI] -
121. Zhou K, Hu X, Chen G, Xu D, Xu X. Circularly polarized photodetectors based on chiral moiré stacking films of electrospun ZnO nanowires. ACS Appl Nano Mater. 2025;8(1):376-383.
[DOI] -
122. Deng W, Lei H, Zhang X, Sheng F, Shi J, Zhang X, et al. Scalable growth of organic single-crystal films via an orientation filter funnel for high-performance transistors with excellent uniformity. Adv Mater. 2022;34(13):2109818.
[DOI] -
123. Zhang X, Deng W, Lu B, Fang X, Zhang X, Jie J. Fast deposition of an ultrathin, highly crystalline organic semiconductor film for high-performance transistors. Nanoscale Horiz. 2020;5(7):1096-1105.
[DOI] -
124. Wen Y, Liu Y, Guo Y, Yu G, Hu W. Experimental techniques for the fabrication and characterization of organic thin films for field-effect transistors. Chem Rev. 2011;11(5):3358-3406.
[DOI]
Copyright
© The Author(s) 2025. This is an Open Access article licensed under a Creative Commons Attribution 4.0 International License (https://creativecommons.org/licenses/by/4.0/), which permits unrestricted use, sharing, adaptation, distribution and reproduction in any medium or format, for any purpose, even commercially, as long as you give appropriate credit to the original author(s) and the source, provide a link to the Creative Commons license, and indicate if changes were made.
Publisher’s Note
Share And Cite