Abstract
Supercapacitors, renowned for their high-power density, rapid charge/discharge capabilities, and exceptional cycling stability, have emerged as promising solutions for sustainable and efficient energy storage. Among various electrode materials, carbon materials stands out due to its abundance, excellent electrical conductivity, chemical stability and structural versatility. This review explores the design strategies, performance optimization, and the expanding applications of carbon-based electrodes for supercapacitors. We first analyze the key factors that impact the performance of carbon electrodes for supercapacitors, including pore structure, surface chemistry, electrical conductivity and nanoscale architecture. Subsequently, we provide an in-depth analysis of recent advancements in the rational design of carbon materials, focusing on strategies for optimizing pore architecture, functionalizing surfaces, enhancing conductivity and designing nanostructures. By addressing performance limitations, the review highlights strategies that have significantly improved the efficiency of carbon electrodes. Furthermore, we explore the practical applications of carbon-based supercapacitors in wearable electronics, self-powered devices, and implantable systems. Lastly, we discuss the challenges and opportunities associated by carbon-based electrodes from the perspective of electrode design and practical application.
Keywords
1. Introduction
The growing global demand for green renewable energy and high-performance energy storage systems has driven extensive research into smart materials for electrochemical energy storage devices, including supercapacitors and metal-ion batteries[1-3]. While lithium-ion batteries currently dominate the market for portable electronics and electric vehicles, their limitations, such as short cycle life, thermal instability, and the dwindling availability of lithium, pose significant challenges. These concerns have accelerated the pursuit of next-generation energy storage systems, with supercapacitors emerging as a promising alternative for more sustainable energy solutions. Positioned between traditional capacitors and batteries, supercapacitors are renowned for their exceptional power density, rapid charge/discharge capabilities, and remarkable cycling stability. These characteristics make them highly suitable for a wide range of applications, from portable electronics to renewable energy systems[4,5]. However, despite their impressive performance, supercapacitors still face critical challenges, particularly in terms of energy density and material optimization.
The performance of supercapacitors is largely determined by their electrode materials, which directly influence energy storage capacity, charge-discharge efficiency, and cycle stability. Fundamentally, research on supercapacitors primarily focuses on the development of advanced electrode materials, as optimizing their composition and structure is key to overcoming existing challenges in energy density and material efficiency. Therefore, an in-depth understanding of electrode materials and their structure-performance relationships is crucial for enhancing supercapacitor efficiency and expanding their practical applications. Among the various materials explored, carbon materials, conductive polymers, and metal compounds remain the most widely used electrode materials for supercapacitors. Conductive polymers exhibit excellent supercapacitor performance over a wide potential range (-1 to +1 V), making them suitable candidates for both positive and negative electrodes. However, conductive polymer electrodes may experience expansion/contraction during ion intercalation/deintercalation processes, leading to mechanical degradation of the electrodes and poor cycling stability[6,7]. Similarly, mono- and binary metal oxides/hydroxides, metal sulfides, and metal phosphides offer higher energy density due to their multiple oxidation states[8,9]. However, their structural instability under repeated charge-discharge cycles results in rapid capacity fading. Moreover, their limited voltage window necessitates integration with other materials, such as carbon-based structures, to enhance electrochemical performance and stability. In contrast, carbon materials are particularly attractive for supercapacitors due to their abundance, high electrical conductivity, excellent chemical stability, and adaptability to various operating conditions. They also exhibit a broad range of allotropes, morphologies, and architecture, spanning from zero-dimensional (0D) to three-dimensional (3D) structures. Their versatility is further enhanced by their ability to exist in various physical forms, including powders, fibers, foams, fabrics, and composites, making them highly suitable for supercapacitor applications[10-13]. The performance of carbon electrode depends not only on the surface properties but also on bulk characteristics. Key factors affecting their efficiency include their specific surface area, pore size distribution, electrical conductivity, morphology, structural architecture, and surface chemistry[13-15]. In particular, during rapid charge-discharge cycles, limited electrolyte ion penetration into the bulk of the carbon material can reduce the effective utilization of available surface area. The structure properties of carbon materials play a crucial role in determining their electrochemical performances. For instance, the pore structure directly affects ion transport and charge storage, with well-optimized porosity enabling faster ion diffusion and higher capacitance[16,17]. Surface chemistry, particularly the incorporation of functional groups, can enhance capacitance through pseudocapacitive interactions and wettability improvement[18]. Electrical conductivity ensures efficient charge transfer within the electrode material, minimizing resistive losses and enhancing rate performance and power density. Additionally, carefully designed nanostructures maximize active surface area, optimize material stability, and enhance the overall electrochemical performances[19,20]. Numerous studies have been dedicated to developing high-quality carbon electrodes with various morphologies/architectures, such as spheres, nanofibers, sheet-like structures and carbon aerogels[21-25]. These studies mainly focus on preparation techniques, nanostructural modifications, and hybridization with psuedocapacitive/battery-type materials. However, a systematic analysis of structure-performance relationship, particularly how specific structural characteristics such as pore architecture, surface chemistry, and nanoarchitecture influence supercapacitor performance and the corresponding strategies to enhance these properties, remains insufficient. Addressing this gap is essential for optimizing material design and guiding future advancements in high-performance carbon-based supercapacitors.
In this review, we aim to fill this knowledge gap by providing a systematic analysis of the structure-performance relationship in carbon electrodes for supercapacitors. We delve into the fundamental principles underlying these critical factors, including pore structure, surface chemistry, electrical conductivity, and nanoscale architecture, as well as their impact on the performance of carbon electrodes in supercapacitors, as depicted in Figure 1. We provide an in-depth discussion of recent advancements in the rational design of carbon materials, focusing on optimizing pore architectures, engineering surface chemistry, enhancing electrical conductivity, and utilizing nanostructure innovations. By integrating findings from recent research, we propose a comprehensive framework that establishes a correlation between material properties and electrochemical performance, thereby providing critical insights into potential strategies for addressing current limitations. Through a detailed examination of state-of-the-art research, we highlight strategies that have successfully addressed performance bottlenecks and improved the overall efficiency of carbon-based supercapacitors. Finally, we explore the applications of carbon-based supercapacitors and discuss their practical applications in wearable electronics, self-powered devices and implantable devices. By bridging fundamental materials science with practical applications, this review provides valuable insights to accelerate the development of next-generation carbon-based supercapacitor materials, paving the way for more efficient and sustainable smart energy storage solutions.
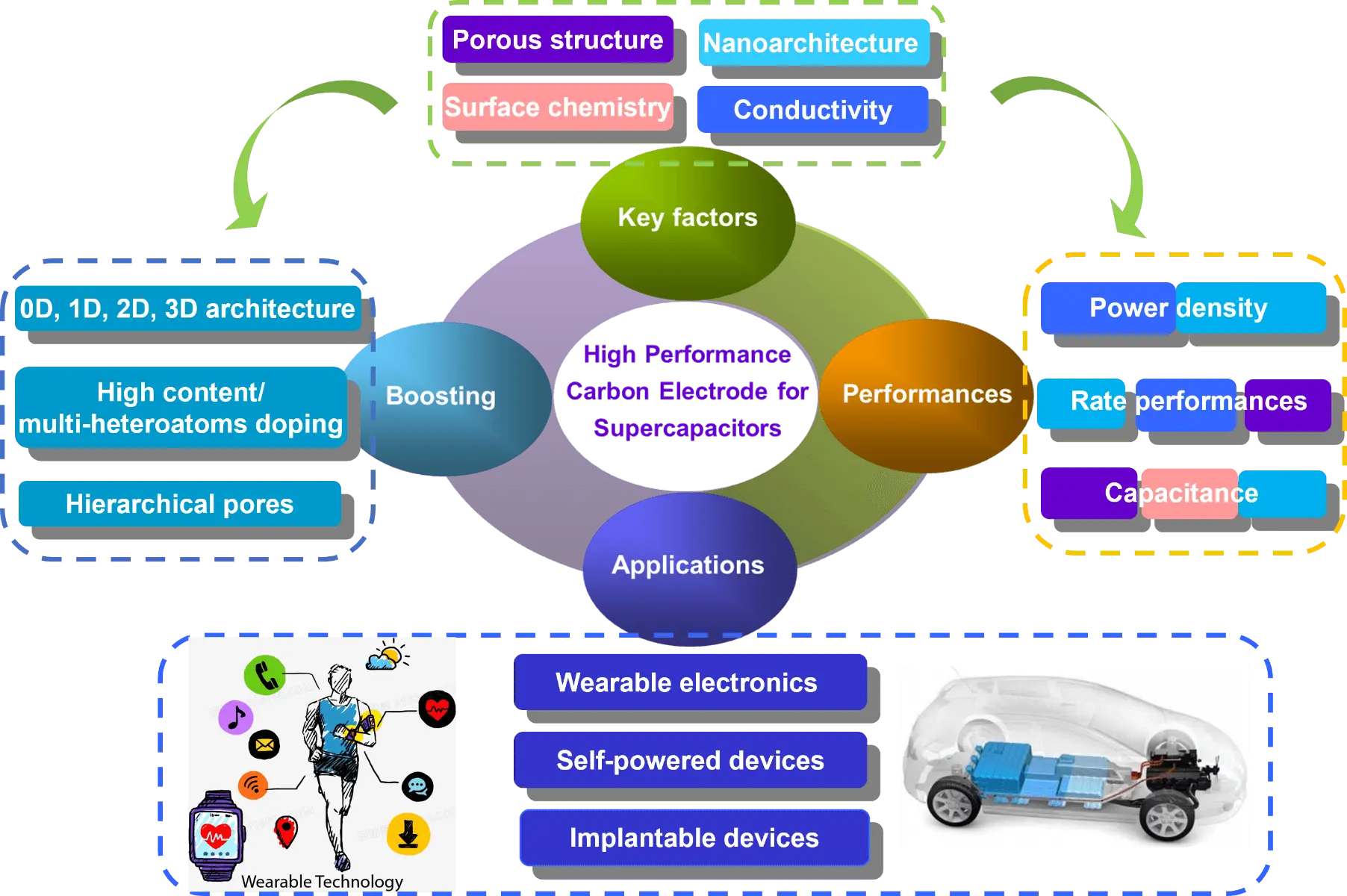
Figure 1. Illustration of the structure-performance relationship and their practical applications of carbon-based supercapacitors. 0D: zero-dimensional; 1D: one-dimensional; 2D: two-dimensional; 3D: three-dimensional.
2. Charge Storage Mechanism of Supercapacitors
Supercapacitors store energy through two primary mechanisms: reversible ion adsorption at the interface between the electrode material and the electrolyte (a purely physical process) or rapid and reversible Faradaic redox reactions occurring on the electrode surface. Based on their energy storage mechanisms, supercapacitors are classified into three types: (1) electric double-layer capacitors (EDLCs), (2) pseudocapacitors, and (3) hybrid capacitors. As shown in Figure 2a,b,c, the electrode materials for supercapacitors are correspondingly divided into three categories: electric double-layer materials, pseudocapacitive materials, and battery-like materials[26].
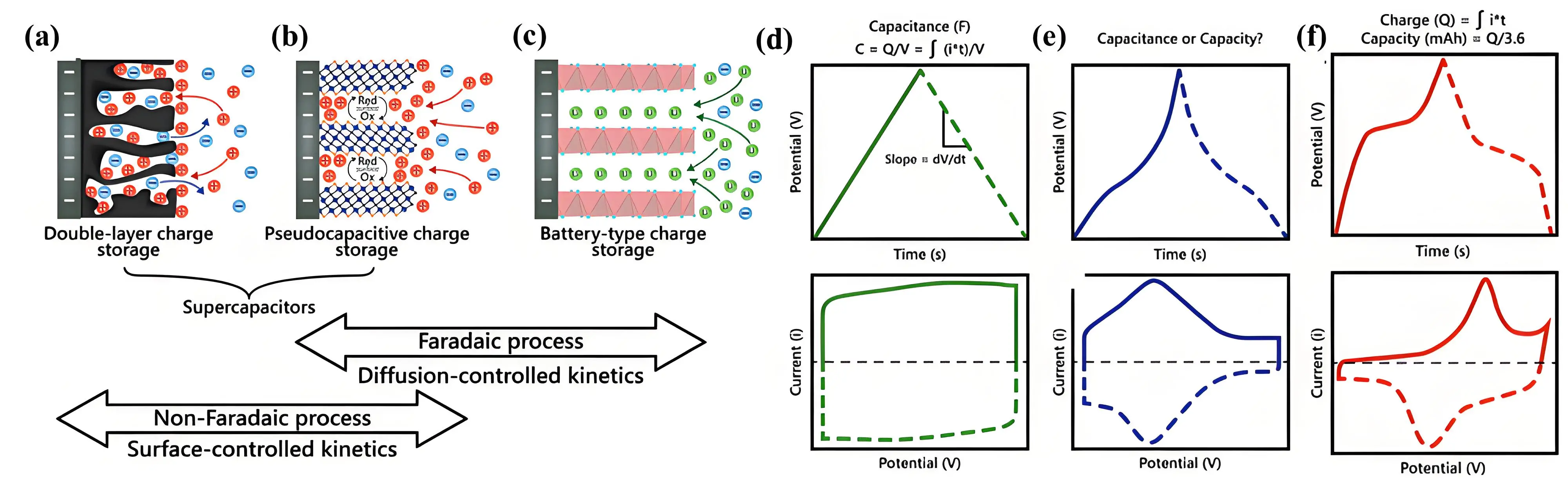
Figure 2. Depiction of the electrode reactions taking place at (a) EDLCs; (b) pseudocapacitive; (c) faradaic electrode. CV and GCD curves of electrodes with different energy storage mechanisms (d) EDLCs electrode; (e) pseudocapacitive electrode; (f) battery-like electrode. Republished with permission from[26]. EDLCs: electric double-layer capacitors; CV: cyclic voltammetry; GCD: galvanostatic charge-discharge.
2.1 EDLCs
In EDLCs, charge storage occurs via reversible ion adsorption and desorption processes at the electrode-electrolyte interface. This is a purely physical process that does not involve chemical reactions. Due to this characteristic, EDLCs exhibit higher cycling stability and power density compared to other types of energy storage devices[27]. During charging, oppositely charged ions from the electrolyte are adsorbed onto the electrode surface, forming a double layer at the interface. The performance of EDLCs is largely influenced by the accessible surface area at the electrode/electrolyte interface. Carbon-based materials, including activated carbon[28], graphene[29], carbon nanotubes (CNTs)[30], and carbon fibers[31] are widely used as electrode materials due to their high specific surface areas and structural stability. However, since the process does not involve Faradaic reactions, EDLCs suffer from the drawback of relatively low energy density.
2.2 Pseudocapacitors
Regarding pseudocapacitors, energy storage occurs through rapid and reversible Faradaic reactions (redox reactions) when a voltage is applied, similar to the charge/discharge process in batteries, but without phase transitions in the electrode material[4,31]. Faradaic reactions not only expand the operating voltage but also increase capacitance. Since energy storage occurs at the electrode surfaces, pseudocapacitors offer much higher capacitance and energy density compared to EDLCs. However, the Faradaic reaction process is generally slower than the physical process of charge adsorption/desorption, which leads to a reduced power density for pseudocapacitors. Additionally, since the energy storage process occurs on the electrode surface, it often leads to material degradation and lower cycling stability[24]. Transition metal oxides, hydroxides, and sulfide capable of redox reactions are commonly used as electrode materials for pseudocapacitors[32,33]. Recent studies have classified the phase-changing energy storage behavior of electrode materials during redox reactions as battery-type capacitance[34].
2.3 Hybrid capacitors
Although batteries and supercapacitors store energy through different electrochemical behaviors, their structural composition is similar, making hybrid battery-supercapacitor devices feasible. Typically, a hybrid supercapacitor combines capacitor-type cathodes and battery-type anodes, therefore, it is promising to integrate the high energy density of batteries with the high-power density and long cycle life of supercapacitors[35,36]. Metal-ion capacitors (lithium, sodium, potassium)[37-40], with a high voltage window of up to 4.5 V, have been extensively investigated. However, the large radius of sodium ions and potassium ions may result in a slower ion diffusion rate. Moreover, hybrid ion capacitors face difficulties in simultaneously achieving high energy density and high power density, primarily due to the mismatch in capacity and kinetics between the anode and cathode materials.
The cyclic voltammetry (CV) and galvanostatic charge-discharge (GCD) curves provide insight into the performance of the three types of electrode materials. For materials exhibiting a typical electrical double-layer response, the voltage and time on the GCD curve show a linear relationship, resulting in a triangular-shaped GCD curve while the CV curve appears rectangular (Figure 2d). In contrast, for pseudocapacitive and battery-like materials, the GCD curves exhibit a nonlinear voltage-time relationship and their CV curves display redox peaks (Figure 2e,f). Especially for the battery-like materials, a distinct plateau appears in the GCD curves.
3. Critical Factors Affecting the Performance of Carbon Electrode Materials in Supercapacitors
Features of carbon materials, like porous architecture, electrical conductivity, surface chemistry, and nanoscale design are crucial in determining their electrochemical performance. These factors collectively play a vital role in achieving high capacitance, high-rate performance and energy density. The following section provides a detailed analysis of these aspects.
3.1 Porous structure
EDLCs store energy through ions adsorption on the surface of active materials, forming an electric double layer. The pore size and porous architecture of carbon electrode materials play a crucial role in determining the contact area with the electrolyte, directly impacting their specific capacitance and energy density[41]. Chmioja et al. explored the relationship between pore size and capacitance in porous carbon electrodes[42]. As shown in Figure 3a, the normalized capacitance decreases as the pore size approaches 1 nm. However, when the pore size falls below 1 nm, the trend reverses, leading to a pronounced increase in capacitance as the pore size decreases. This finding highlights the importance of subnanometer pores in enhancing specific capacitance. When the pore size significantly exceeds twice the solvated ions diameter, compact ion layers form along the opposing walls of the pore, maintaining capacitance despite the absence of a diffuse charge layer. As pore size decreases to just below twice the ion size, capacitance declines due to overlapping ion layers and reduced usable surface area. However, when pores become smaller than the solvated ion size, capacitance increases sharply due to the distortion of solvation shells, allowing ions to approach the electrode surface more closely and enhancing charge storage. Molecular dynamics simulations of microporous carbons provide an alternative perspective, revealing that smaller pore sizes are advantageous for charge storage. Liu et al. demonstrated that while charging dynamics are constrained by the pore-limiting diameter, equilibrium capacitance is not strongly linked to geometric properties like pore size[43]. Instead, capacitance strongly correlates with the charge compensation per carbon, a newly defined parameter representing the average charge of electrode atoms surrounding a counterion. Higher charge compensation per carbon values indicate more efficient charge storage, as stronger partial charges allow for greater ion adsorption (Figure 3b). These high charge compensation per carbon adsorption sites are often found in areas with smaller curvature radii, allowing counterions to interact more effectively with multiple sites and further enhancing charge storage.
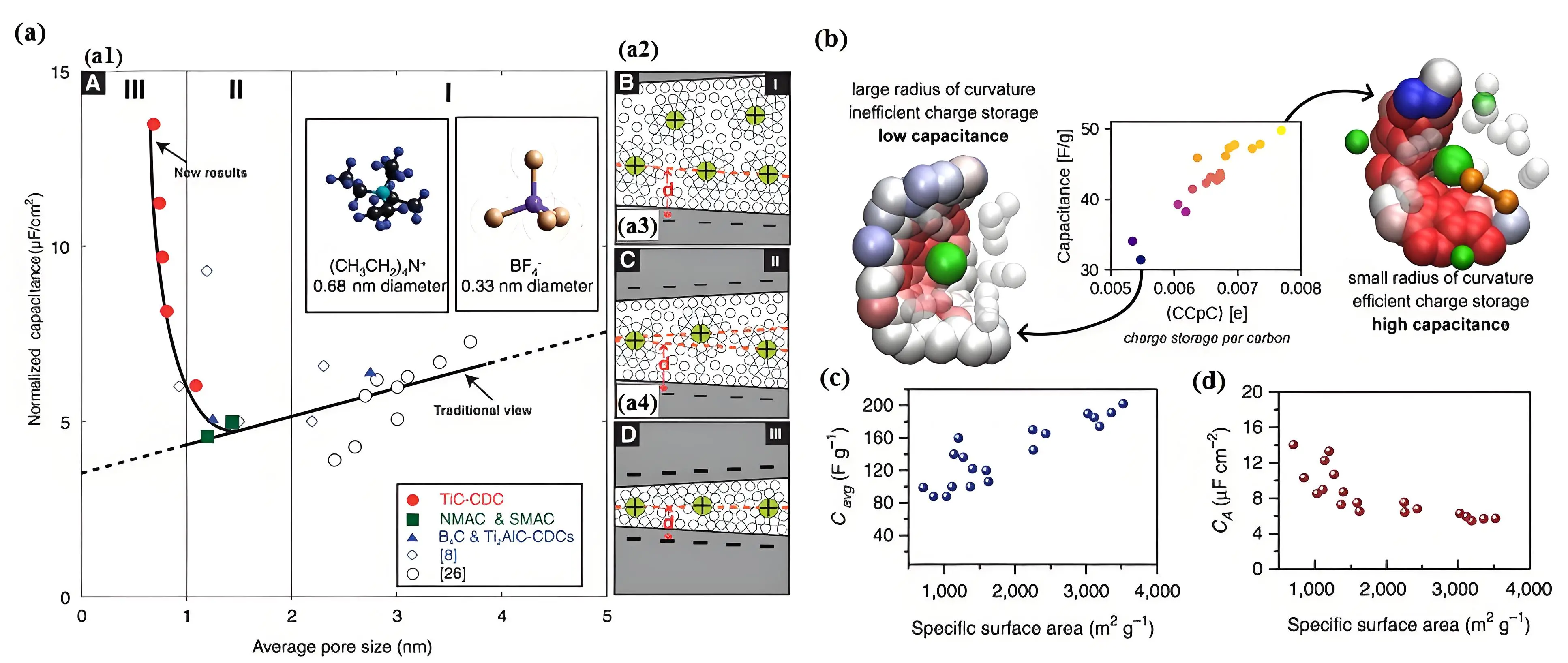
Figure 3. (a) Specific capacitance normalized by pore size for the carbons. Rrepublished with permission from[42]; (b) charge storage per carbon at varying curvature radii[43]; (c) gravimetric; (d) areal capacitance versus the specific surface area[27]. CDC: carbide-derived carbons; NMAC: natural material precursor activated carbon; SMAC: synthetic material precursor activated carbon; CCpC: charge compensation per carbon.
In particular, regarding aqueous electrolyte, density functional theory (DFT) calculations reveal that the pore diameters centered around 0.7-0.8 nm and 1-2 nm are ideally suited for accommodating hydrated K+ and H+ ions[44,45]. Ultramicropores smaller than 0.5 nm significantly hinder the efficient utilization of surface area, as aqueous electrolyte ions are unable to access these tiny pores, ultimately limiting the energy storage potential of EDLCs. Meanwhile, for organic electrolytes, mesoporous-scale pore sizes are required. The influence of pore regularity and morphology on ion-transfer resistance is less pronounced in aqueous systems compared to organic systems. This is primarily due to the higher ionic conductivity of aqueous systems and the smaller sizes of solvated ions in water relative to those in organic electrolytes[46]. In other words, the specific capacitance of porous carbon electrodes is mainly influenced by the specific surface area of large micropores and small mesopores[47]. Thus, a combination of abundant micropore larger than 0.5 nm and mesopores is crucial for achieving a high accessible surface area, which is essential for an improving specific capacitance. Wei et al. synthesized hierarchical porous carbon with pore sizes ranging from 0.5 to 4 nm, which are well-matched to the dimensions of solvated K+, H3O+, and sulfate ions[48]. This design greatly enhanced the specific capacitance (400 F g-1 at 1 A g-1 in 6 M KOH) and ensured efficient ion transport, with 62% capacitance retention at 30 A g-1. Additionally, it achieved a high energy density of 11.05 Wh Kg-1 at a power density of 5,368 W kg-1. Zhang et al. tuned the porous structure of biomass-derived carbons and highlighted the importance of a balanced pore structure for achieving high electrochemical performance[49]. They revealed that a high micropore density increases surface area, an excess of micropores hinder mass transport. An ideal pore structure with a micro-to-mesopore ratio of 2.38 optimizes the active surface area and creates efficient mass transport channels, resulting in high capacitance (447 F g-1 at 0.5 A g-1), energy density (11.2 Wh kg-1), and power density (3.0 kW kg-1). For graphene materials, the capacitance is directly proportional to the specific surface area, with gravimetric capacitance increasing as the surface area expands, while the areal capacitance decreases with the specific surface area (Figure 3c,d)[27]. Optimizing the pore architecture of carbon materials is crucial for improving electrochemical storage performance. While large micropores and small mesopores may enhance energy density, larger pore sizes may be more advantageous for power density. Further tuning of the pore structure in carbon materials, specifically by designing carbon with numerous narrow and short pores, can simultaneously improve both energy and power performance.
3.2 Surface chemistry
Heteroatom doping is a well-established strategy to enhance the surface properties of porous carbon materials by modifying their structural and electronic characteristics. For instance, the incorporation of heteroatoms can significantly improve the conductivity of these materials. Additionally, heteroatom doping offers synergistic benefits, such as enhancing electrode stability and wettability, facilitating Faradaic reactions on the carbon surface, and contributing to additional pseudocapacitive behavior. It is widely acknowledged that micropores in carbon materials cannot be fully wetted in aqueous electrolytes, limiting their accessibility and utilization. Oxygen functional groups can enhance the wettability of micropores within carbon electrodes, thereby increasing specific capacitance. Figure 4a shows the possible oxygen (O) and nitrogen (N) functional groups in carbon framework. Among these groups, phenolic hydroxyl and carbonyl groups positively impact capacitance, while carboxyl groups, being structurally large and unstable, impede electrolyte migration within the pores and are less effective in boosting overall capacitance[50].
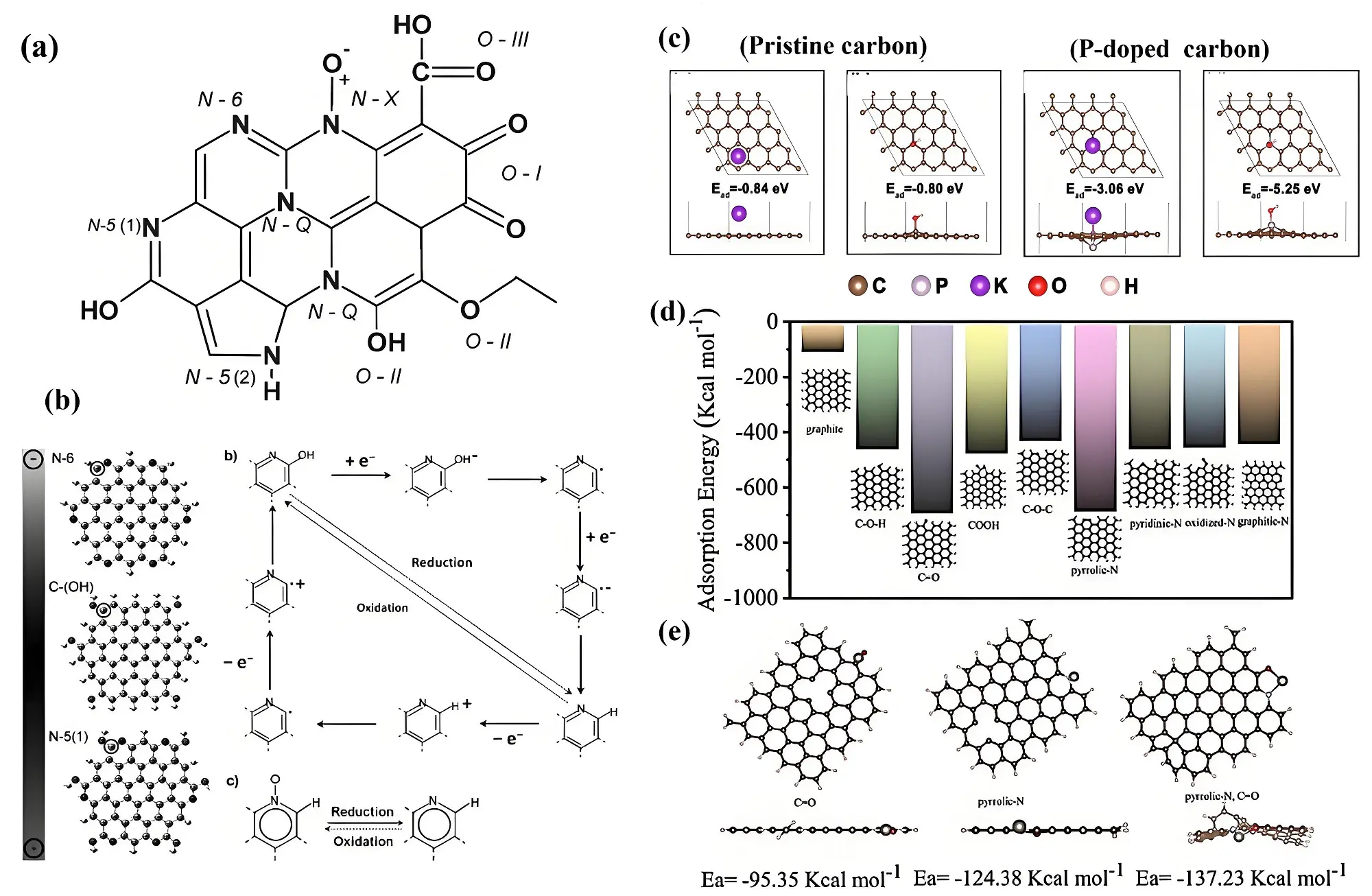
Figure 4. (a) Surface O and N functional groups in carbon materials; (b) the proposed faradic reactions of nitrogen groups. Republished with permission from[52]; (c) adsorption energy of K+ and OH- in pristine carbon and P-doped carbon[54]; (d) cluster models of various heteroatom-doped carbons and (e) their corresponding adsorption energies for Zn2+[56].
Faradaic reactions primarily occur at the edges of carbons materials. Nitrogen, with five valence electrons, acts as an electron donor within the lattice, leading to a shift of the Fermi level closer to the valence band in carbon electrodes, thereby improving conductivity within the carbon skeleton. Pyridone nitrogen and pyridinic nitrogen atoms stand out as the most influential, as they contribute significantly to capacitance through faradic reactions in both acidic and alkaline electrolytes[51,52]. Pyridone nitrogen atoms can be viewed as pyridine nitrogen atoms with a neighboring carbon atom bonded to a hydroxyl group, allowing for interconversion between pyridone and pyridine nitrogen atoms during electrochemical processes. Pyrrolic nitrogen is found in a five-membered ring with one carbon replaced by nitrogen, and its conversion to a six-membered ring occurs only at high temperatures. As depicted in Figure 4b, during reduction, pyridone nitrogen and pyridinic nitrogen are converted into N-6 and N-Q, while oxidation reverses the process, regenerating pyridone nitrogen and pyridinic nitrogen[52]. Although N-Q groups do not participate in Faradaic reactions, they enhance capacitance by introducing positive charges, which facilitate improved electron transfer[51]. The redox reaction mechanism of co-doped nitrogen and oxygen atoms is believed to involve attracting protons or enhancing the charge density of the space charge layer, thereby promoting the oxidation/reduction of quinones and introducing redox activity from amine groups[44,53].
On the other hand, phosphorus (P) atoms can contribute to the stabilization of oxygen functional groups during electrochemical charging and introduce additional active sites for ion adsorption thereby enhancing electrochemical performance. As shown in Figure 4c, DFT calculations were also used to investigate the effects of P-doping[54]. The results indicate that the adsorption energies of K+ and OH- on pristine carbon surfaces are relatively low, at -0.84 eV and -0.80 eV, respectively, leading to unstable adsorption. In contrast, the adsorption energy of K+ significantly increases to -3.06 eV, and for OH-, it rises to -5.25 eV on P-doped carbon surfaces, demonstrating strong adsorption capability. Boron (B) atoms are more challenging to incorporate into the carbon lattice than nitrogen atoms. Unlike N, which can easily interact with the stable carbon atoms in the six-membered ring, B tends to bond more readily with reactive N atoms that have unpaired electrons. The B-N bonding configuration is thermodynamically more stable compared to B-C and C-N bonds. That is to say, the introduction of B, with its electron-deficient outer shell, can stabilize active nitrogen species in the carbon lattice by coupling with nitrogen, preventing their escape or conversion to inert forms. Sun et al. synthesized B and N co-doped carbon materials with impressive conductivity (30 S cm-1) and a high nitrogen content (7.80 at%)[55]. DFT simulations revealed that B lowers the energy levels of neighboring nitrogen species, particularly pyrrolic nitrogen, which enhances their stability and boosts electrochemical performance. As a result, the nitrogen-rich porous carbon electrode exhibited a remarkable capacitance of 412 F g-1 at 1 A g-1 in 1.0 M H2SO4 and high durability with 97% capacitance retention after 10,000 cycles.
Regarding Zn-ion supercapacitors, Chu et al. examined the adsorption energies of zinc ions on both unmodified and functionalized carbon surfaces, revealing that the introduction of N and O functional groups significantly enhances zinc ion affinity compared to unmodified carbon (Figure 4d)[56]. Among the functional groups, C=O and pyrrolic-N exhibited the highest adsorption energies, measured at -694.11 kcal/mol and -687.28 kcal/mol, respectively. Furthermore, simulations demonstrated that O-doped, N-doped, and O, N co-doped carbon surfaces interact with Zn2+ ions with adsorption energies of -95.35 kcal/mol, -124.38 kcal/mol, and -137.23 kcal/mol, respectively (Figure 4e). These findings highlight the critical role of C=O and pyrrolic-N groups in enhancing Zn2+ ion adsorption, offering valuable insights for optimizing functionalized carbon materials.
3.3 Conductivity
As electrode materials for supercapacitors, high conductivity plays a crucial role in reducing internal resistance (ohmic loss), promoting electron transport, and minimizing both internal and charge transfer resistance within the electrodes. This is especially crucial for rate capability and high power density, where rapid charge and discharge are required. Moreover, while energy density primarily depends on specific capacitance and operating voltage, high conductivity is essential to ensure the efficient utilization of the active area by facilitating the rapid transport of ions and electrons, thereby indirectly enhancing energy storage efficiency.
Traditional porous carbon materials usually have electrical conductivity lower than one siemens per meter (S m-1). The porosity and surface properties of carbon materials can significantly affect the continuity and efficiency of conductive pathways. Controlling porosity and pore size distribution, such as reducing excessive micropores or dead pores and increasing accessible mesopores and macropores can enhance the connectivity of electronic pathways[57,58]. High-temperature (> 2,500 °C) graphitization can effectively improve the ordering of carbon atoms, leading to increased graphitization and conductivity[21,59]. The development of well-ordered graphitic crystalline regions within nanostructures is crucial for the efficient transport of electrons and ions. However, high carbonization temperatures can lead to pore collapse, resulting in a decrease in surface area and pore volume. Removing surface defects or excessive oxygen-containing groups (e.g., -COOH or -OH) reduces the hindrance to electron migration. For example, removing the oxygen functional groups on graphene oxide endows the graphene fibers with excellent electrical conductivity of 1.06 × 105 S m-1[60].
The introduction of heteroatoms (such as N, S, B, or P dopants) can modulate the concentration and mobility of electrons, thereby enhancing conductivity[61]. For example, N-doping improves electron transfer capabilities, creates asymmetric charge distribution, and modifies spin density due to its high electronegativity (3.04). Sulfur (S) doping, with its electronegativity (2.58) close to that of carbon (2.55), adjusts the electronic configuration of the carbon surface and the graphitic lattice[62]. In contrast, P atoms, with a larger atomic radius and lower electronegativity (2.19), distort the carbon lattice and introduce electrons into the structure[63]. Numerous studies have focused on single- and multi-heteroatoms doped carbon materials. It has been revealed that the electronic interaction between N and B optimizes the conductivity of the material, accelerating charge transfer kinetics[64,65]. One-dimensional (1D) and two-dimensional (2D) carbon architectures (such as CNTs and graphene) typically exhibit excellent electrical conductivity due to their extended electron transport pathways and unique electronic structures. Liu et al. found that phosphorus-doped CNTs, offering excellent electrical conductivity and an interconnected porous structure, facilitate efficient electron transfer from electroactive materials to external circuits[66]. The high conductivity of P-doped CNTs also enables the rapid transport of electrons generated within the electroactive components. As a result, the designed self-supporting film achieves enhanced specific capacitance.
3.4 Nanoarchitecture
The dimensional structure of carbon materials is a key factor in determining their performance in supercapacitors, as it affects properties like specific surface area, pore structure, and electrical conductivity. For instance, 0D carbon materials, such as carbon/graphene quantum dots and carbon nanospheres, have shown great promise in supercapacitors due to their exceptional electrical properties and structural benefits. These include small size, excellent conductivity, large surface area, numerous active sites, and superior solubility in various solvents. In particular, the ultrasmall dimensions of quantum dots grant them exceptional electron transfer and storage capabilities, along with enhanced edge quantum effects[67,68]. 1D carbon materials, such as CNTs, carbon nanofibers and graphene fibers, combine the effects of micro- and nano-scales, providing pathways for rapid ion and electron transport along their axes[69,70]. 2D carbon materials, such as graphene, reduced graphene oxide, and carbon nanosheets, feature a layered structure that offers a large accessible surface area for charge storage. Their excellent in-plane electrical conductivity facilitates rapid electron and ion transport, while also ensuring efficient electrolyte access. This structure enhances ion adsorption, boosts capacitance, and improves rate performance by enabling inherently fast electron and ion diffusion dynamics[71,72]. 3D carbon materials (e.g., porous carbon materials, carbon aerogels, and carbon foams) characterized by interconnected porous channels and high mechanical strength, can withstand stress changes, facilitate substantial contact between the electrode and electrolyte, and improve electronic conductivity, significantly boosting the overall electrochemical performance[58,73]. The design of nanostructures to boost the performance of carbon materials will be systematically discussed in the following sections.
4. Strategies for Enhancing the Performances of Carbon Electrode Materials
4.1 Pore structure engineering
As outlined in Section 3.1, micropores and small mesopores are essential for enhancing capacitive performance, as they provide a large accessible surface area for ion transport and charge storage. On the other hand, larger mesopores and macropores function as ion reservoirs, helping to shorten the diffusion distance for ions to penetrate the material's interior. This is particularly important for maintaining capacitance at high current densities and boosting power density. As depicted in Figure 5a, Wen et al. explored the relationship between the diffusion path and diffusion time, and revealed that abundant mesopores improved the diffusion coefficients of SO₄2- ions in lignin-derived porous carbons. The diffusion coefficients of electrodes carbonized at different temperatures were 2.53 × 10-9 m2 s-1, 8.41 × 10-10 m2 s-1, and 7.46 × 10-10 m2 s-1, respectively. These values are about 10 times higher than that of commercial activated carbon (4.65 × 10-11 m2 s-1)[74].
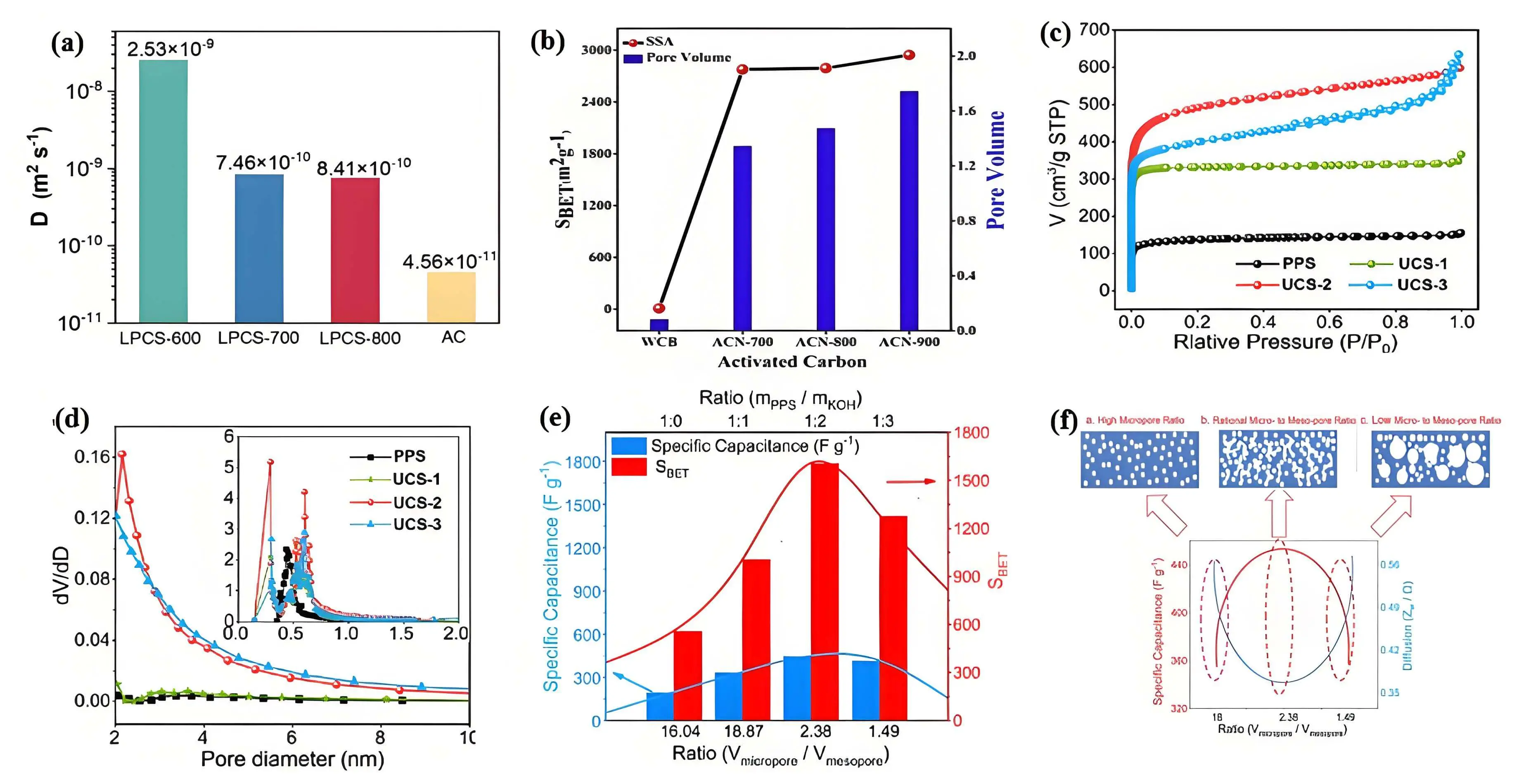
Figure 5. (a) Diffusion coefficient of SO42- in cathodes base on mesopore dominated hierarchical porous carbon and activated carbon electrode[74]; (b) surface area and total pore volume of juliflora wood waste-based carbon[16]; (c) nitrogen sorption isotherms; (d) pore size distribution curves of KOH-activated carbon; (e) relationship between specific capacitance, the micro-to-meso-pore ratio, and the mass ratio of hemicellulose to KOH; (f) impact of pore structure on the electrochemical performance[49].
Hierarchically porous carbons, which integrate both microporous and mesoporous structures, achieve an optimal balance between energy density and power density. This design outperforms carbons with a single pore size distribution (microporous, mesoporous, or macroporous). The synergy between pore sizes enhances ion transport efficiency while maximizing charge storage capacity. Numerous fabrication techniques have been developed to create hierarchical porous carbon architectures. Physical activation (e.g., CO2, H2O) or chemical activation (KOH, ZnCl2, CaCl2, ZnC2O4, Cu(NO3)2) is commonly employed to enhance specific surface areas and tune the pore structure, especially for the porous carbons derived from biomass and industrial polymers[75-77]. For physical activation, the gases usually react with carbon to create micropores by etching the carbon framework at high temperatures. This method produces carbon materials with excellent electrical conductivity and outstanding cycling stability; however, the limited control over pore size distribution often leads to excessive microporosity, which can hinder ion transport and reduce rate performance. Regarding chemical activation, activating agents react with carbon precursors at lower temperatures to create pores and enhance the formation of hierarchical structures, allowing for better control over micropore and mesopore formation. This enhances charge storage capacity and rate capability, but the introduction of heteroatoms functional groups may slightly reduce electrical conductivity, and the durability is generally lower than that of physically activated carbon materials. The activation process, which involves factors such as the amount of activator used and the activation temperature, can have a significant impact on the internal pore structure of the resulting carbon material. For example, when the pyrolysis temperature remains below the boiling point of ZnCl2 (732 °C), the activation process predominantly generates micropores. Increasing the concentration of ZnCl2 tends to promote the formation of larger mesopores or macropores. After activation, carbon materials typically exhibit a surface area exceeding 2,000 m2 g-1 and a pore volume larger than 1.0 cm3 g-1. However, excessively high surface areas can lead to drawbacks, such as increased vulnerability to electrolyte decomposition at dangling bond sites, decreased material density, and reduced conductivity. Furthermore, the sieving effect of electrolyte ions may limit ion accessibility. Therefore, optimizing pore size and distribution is more crucial than simply maximizing surface area to achieve the highest capacitance[44]. Raja et al. activated prosopis juliflora wood waste carbon blocks using KOH at varying pyrolysis temperatures (700-900 °C)[16]. Increasing the activation temperature significantly increased the content of mesopores and pore volume, but it has little impact on the specific surface area (Figure 5b). Wei et al. investigated the distinct roles of mesopores and micropores (Figure 5c,d,e). They adjusted the micropore-to-mesopore ratio by tuning the weight ratio of partially dissolving hemicellulose to KOH. For example, when the mass ratio of pre-carbonized pecan shell to KOH was 1:2, the resulting carbon (US-2), with a micropore-to-mesopore ratio of 2.38, demonstrated the highest specific surface area of 1,785.6 m2 g-1 and an impressive specific capacitance of 447 F g-1 at 0.5 A g-1 in a 6 M KOH electrolyte, which remained 62.6% at 50 A g-1[49]. Recently, milder alternatives, such as gas-releasing agents (e.g., ammonium bicarbonate, zinc acetate, potassium acetate), were also used to fabricate hierarchical porous carbons[78]. Feng et al. employed three types of pore-forming agents (K2CO3, SiO2, and ZnC2O4) to fabricate lignin-derived porous carbons and investigated their impact on nanoporous structures[77]. It was found that the hierarchical porous structure, consisting of nanosheets formed via ZnC2O4 activation, offered superior charge storage and ion transport capabilities compared to the microporous structure created with K2CO3 and the mesoporous structure produced using the SiO2 template method.
Template-assisted methods (e.g., silica, zeolite) have proven highly effective for designing tailored pore structures[79]. The removal of the template generates numerous pores in the carbon material. However, due to the uniformity of templated pore structures, multiple templates are often needed to achieve hierarchical porous carbons. In addition to pore structure and specific surface area, pore volume is another critical factor that significantly influences the rate capability and specific capacitance of supercapacitors. The overall pore volume determines the charge adsorption capacity, whereas the mesopore volume influences the electrolyte resistance. A higher pore volume allows for sufficient space for electrolyte ions to effectively penetrate deeper pores. Liu et al. synthesized micro-mesoporous carbon nanospheres featuring a surface area of 1,051 m2 g-1 and an exceptionally large pore volume of 3.684 cm3 g-1[80].The substantial pore volume enables efficient charge storage and enhances electrochemical performance by allowing rapid mass transport. As a result, the hierarchical porous carbon exhibits impressive specific capacitances of 309.4 F g-1 at 0.5 A g-1 in 1 M H2SO4 which remains 75% when the current density increases to 50 A g-1, showcasing the enhanced rate performance. Table 1 summarizes the performance of carbon electrodes with different porous structures. Pore size distribution and specific surface area are two critical factors influencing the performance of carbon materials in supercapacitors. Capacitance is closely linked to the effective surface area, as a higher specific surface area provides more active sites, enhancing charge storage and boosting specific capacitance. However, maximizing the surface area alone is not sufficient to achieve high-performance supercapacitors. Instead, an optimized pore size distribution, particularly a well-balanced combination of micropores, mesopores, and macropores, is essential for improving electrochemical performance. Micropores (> 0.5 nm) contribute to charge storage by providing abundant adsorption sites for electrolyte ions, mesopores facilitate efficient ion transport, and macropores improve ion accessibility and minimize diffusion resistance. A higher mesopore-to-micropore ratio has been demonstrated to significantly enhance the rate capability of electrode materials, primarily due to improved ion diffusion kinetics and lowering transport resistance within the hierarchical porous structure. A well-designed pore architecture ensures high capacitance, excellent rate capability, and long-term cycling stability. By optimizing both pore size distribution and specific surface area, the overall efficiency and practicality of supercapacitor materials can be greatly improved.
Samples | Surface area/m2 g-1 (pore volume/cm3 g-1) | Pore size (nm) | Activation agent | Micro/ mesopores(a) | Electrolyte | Capacitances/F g-1 (at current density/A g-1)(b) | Rate% (current density/A g-1)(b) | Energy density (Wh kg-1)(c) | Power density (kW kg-1)(c) | Device stability% (cycles) | Ref. |
PF24GO-HA | 1,779 (1.78) | 0.5-10 | KOH | 33/67 | EMIMBF4 | 220 (1) | - | - | - | - | [41] |
Ti2AlC-derived carbon | 1,150 | < 1.0 | - | - | 1.5 M TEABF4/AN | 140 (0.5) | - | - | - | - | [42] |
Biomass-derived hierarchical porous carbon | 2,943 (1.83) | 1.3-90 | KOH | - | 6 M KOH | 588 (0.5) | 76.9 (0.5-10) | 32.9 | 0.172 | 94.7 (6,000) | [16] |
N/O doped porous carbons | 761 (0.39) | 0.7, 2-50 | ZnCl2 | 56/44 | 1 M H2SO4 | 370 (0.5) | 80.8 (0.5-30) | 10.3 | 0.12 | 92 (10,000) | [44] |
Sweet potato-derived porous carbon | 3,114 (-) | 1.25, 2.51 | KOH | - | 1M H2SO4 | 532 (1) | 49.6 (1-30) | 25.8-11.9 | 0.25-13.1 | 95.1 (10,000) | [45] |
N-doped porous carbon | 2,675 (1.06) | 0.5-4 | KOH | 91/8 | 6 M KOH | 400 (1) | 62 (1-30) | 11.05 | 5.368 | 90.7 (10,000) | [48] |
Biomass-derived carbon sheets | 1,786 (-) | 0.5-8 | KOH | 69/29 | 6 M KOH | 447 (0.5) | 62.6 (0.5-50) | 15.5-9.7 | 0.062-6.24 | 95/10,000 | [49] |
N-doped hierarchical porous carbon | 892 (0.65) | 1-100 | CaCl2 | 29/71 | 6 M KOH | 275 (0.2) | 81 (0.2-8) | - | - | - | [75] |
Microporous carbon nanofibers | 597 (0.67) | 0.7-1.2 | KOH | 96/4 | 6 M KOH | 256 (0.2) | 67 (0.2-20) | - | - | - | [76] |
N-doped lignin-derived porous carbons | 826 (0.94) | 0.5-100 | ZnC2O4 | 18/62 | 6 M KOH | 254 (0.5) | 79 (0.5-20) | 5.42 | 0.5 | 95.2 (10,000) | [77] |
N doped porous carbon | 2,042 (-) | 0.5-3, 10-20 | K3C6H5O7 Zn(AC)2 | - | 6 M KOH | 447 (0.5) | 64.7 (0.5-10) | 11.25-7.78 | 0.25-5 | 80.4 (10,000) | [78] |
Hierarchical porous carbon nanospheres | 1,051 (3.7) | 0.9, 3.2, 7.7 | - | - | 1 M H2SO4 | 309.4 (0.5) | 75 (0.5-50) | 27.8-20.3 | 0.18-9 | 98.5 (10,000) | [80] |
(a): Percent of pore volume contributed by micropores/mesopores; (b): specific capacitance and rate performance listed were obtained from three-electrode system; (c): energy density and power density listed were obtained from symmetric supercapacitors based on the carbon materials mentioned in the first column.
4.2 High-content and multi-heteroatom-doping
Doping porous carbon materials with heteroatoms (such as N, P, S, and B) can significantly improve their conductivity and hydrophilicity, making them suitable for use in supercapacitors. There are two main methods for introducing heteroatoms into the carbon framework: in situ doping and post-doping. In situ doping involves using heteroatom-containing carbon precursors, catalysts, and other additives to construct the carbon framework. Post-doping involves activating the carbon framework with heteroatom-containing gases or liquids to introduce heteroatoms. Among these, in situ doping is more convenient and generally results in a higher content of heteroatoms compared to post-treatment methods. N is the most commonly used heteroatom for doping. N-doped porous carbon materials can be synthesized using N-containing carbon sources such as polydopamine[81], 3-aminophenol[82], glycine[83], melamine[84] and metal-organic frameworks (MOFs)[85]. In general, the choice of dopant and carbon source affects the uniformity and content of heteroatom doping. Thus, to incorporate more nitrogen into the carbon framework, a carbon precursor with a high nitrogen content is required[86,87]. Wang et al. synthesized porous carbon with tunable nitrogen content using high-nitrogen-content (36 wt%) fumaronitrile as the precursor[87]. The nitrogen doping level (14-34 wt%) and doping types could be modulated by tuning the synthesis temperature. The sample carbonized at 700 °C exhibited a specific capacitance of 400 F g-1 at 1 A g-1 in a 6 M KOH electrolyte. However, studies using CV have shown that nitrogen-doped carbon electrodes do not exhibit distinct redox signals, particularly when tested with alkaline electrolytes[52]. Other heteroatoms like B, S, and P have also demonstrated significant improvements in the performance of porous carbon materials. Since the atomic radii of S and P are larger than that of N, they can create polarized lone pairs of electrons, which further enhance chemical properties and increase defects[54,62,88,89]. Hajibaba et al. synthesized S-doped porous carbon through electrochemical deposition[62]. The high sulfur doping (up to 25%) enhanced the specific capacitance, increasing from 147 to 440 F g-1 at 1 A g-1 after undergoing the electrochemical doping process in 1.0 M H2SO4. Computational analysis based on joint density functional theory demonstrated that introducing S outside the graphene structure significantly boosts the capacitance. The presence of humps around ~ 0.1 and 0.3 V in the S-doped sample suggests pseudocapacitive behavior (Figure 6a), attributed to faradaic redox reactions. P-doping also induced humps in the CV curves (Figure 6b), Zhou et al. revealed that the pseudocapacitive contributions originating from P-doped porous carbon is 22.1% at 5 mV s-1[54].
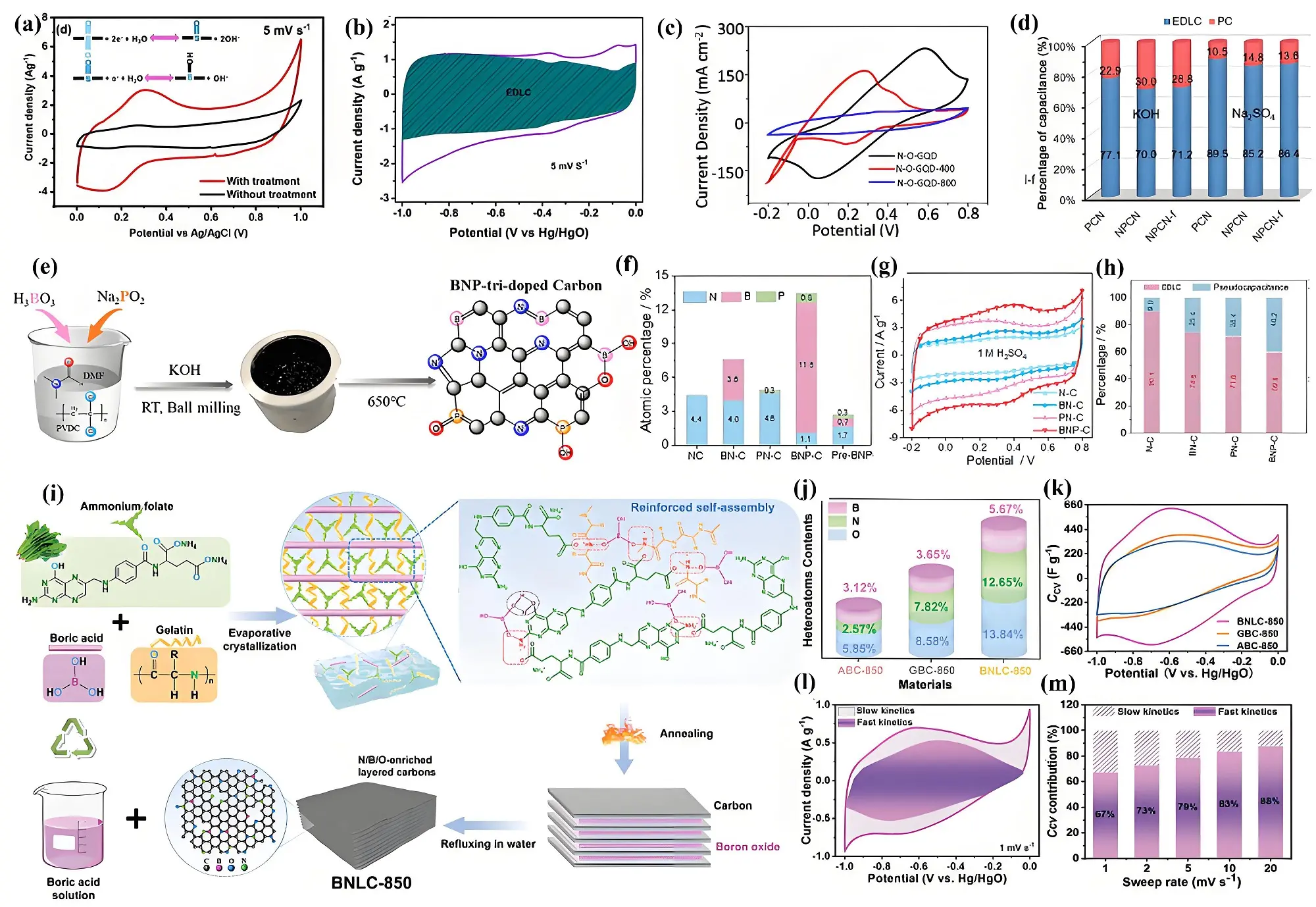
Figure 6. (a) CV curves of S-doped porous carbon at 5 mV s-1 and the possible faradic redox reactions[62]; (b) CV curves of P-doped porous carbon at 5 mV s-1[54]; (c) CV curves of O, N co-doped QCDs[92]; (d) EDLCs and pseduocapacitive contributions of P, N co-doped porous carbon. Republished with permission from[94]; (e) synthesis mechanism; (f) element content analysis; (g) CV curves at 10 mV s-1; (h) capacitance contribution of different heteroatoms doped carbons in 1 M H2SO4[65]; (i) synthesis mechanism; (j) element analysis; (k) CV curves of different heteroatoms doped carbons; (l) CV curves highlighting the diffusion contribution at 1 mV s-1; (m) normalized diffusion contribution across a range of 1-20 mV s-1. Republished with permission from[18]. CV: cyclic voltammetry; QCDs: quantum carbon dots; EDLCs: electric double-layer capacitors.
Compared to doping with a single heteroatom, dual- or multi-heteroatom doping can significantly enhance pseudocapacitance due to synergistic effects[63,90,91]. N and O functional groups significantly enhance the polarity and wettability of the electrode surface, promoting improved electrolyte adsorption and infiltration and thereby contributing to substantial pseudocapacitance. DFT calculations further reveal that N doping lowers the energy barrier for oxidation-state changes in carbonyl groups, thus enhancing pseudocapacitive reactions[74]. Li et al. synthesized graphene quantum dots (GQDs) with ultra-high N and O content (17.8 at.% and 21.3 at.%, respectively) by using pyrene as a precursor, and revealed that the pyrrolic-N and carbonyl components significantly enhance the pseudocapacitive activity of the electrodes[92]. The CV curves of unannealed (N-O-GQD) and low-temperature-annealed (N-O-GQD-400) display a typical pseudocapacitive feature in 1 M H2SO4 (Figure 6c). The GQDs-based supercapacitors exhibit a volumetric specific capacitance of 56.1 F·cm-3 at 50 μA·cm-2 and demonstrate a volumetric energy density of 7.8 mWh·cm-3 at 140.4 mW·cm-3. In addition, S/P can also be co-doped with nitrogen in the carbon framework, creating synergistic effects that further enhance electrochemical performance[93]. Liu et al. developed a self-supporting N,P co-doped carbon electrode using a templating/activating carbonization process combined with vacuum filtration[94]. The introduction of phosphorus (2.1 at.%) and nitrogen (4.1 at.%) heteroatoms significantly enhances the performance by providing abundant active sites. Quantitative analysis revealed that pseudocapacitance contributed 22.9%-30% to the electrochemical behavior under alkaline conditions (Figure 6d).
During carbonization, the nitrogen content generally diminishes with rising temperatures[95]. DFT analysis demonstrates that boron doping reduces the energy levels of neighboring nitrogen species, particularly pyrrolic nitrogen, within the graphene framework, thereby enhancing their stability[55]. Building on this concept, Yu et al. synthesized a ternary B, N, and P co-doped porous carbon material (BNP-C) through a polymer dehalogenation method, achieving atomic compositions of 11.5% B, 1.1% N, and 0.8% P (Figure 6e,f)[65]. Comparative CV analysis at a scan rate of 10 mV s-1 revealed that BNP-C exhibited superior electrochemical performance compared to control samples (BN-C, PN-C, and NC), underscoring the synergistic effects of multi-element co-doping in improving electrochemical activity. Moreover, BNP-C exhibits quasi-rectangular shapes with noticeable redox features between 0.2 and 0.4 V (Figure 6g), confirming the beneficial impact of heteroatom doping in enhancing pseudocapacitance. Accompanied by the 3D hierarchical porous structure and high specific surface area (1,118.5 m2 g-1), BNP-C contribute to a high capacitance of 518 F g-1 at 0.5 A g-1, while pseudocapacitance accounts for approximately 40.2% of the total capacitance as determined by the Trasatti method (Figure 6h). As shown in Figure 6i, Yu et al. developed N/B/O co-doped layered porous carbons (BNLC-850) through carbonizing the framework assembled from ammonium folate, gelatin and boric acid[18]. The N-rich ammonium folate facilitates the formation of a robust 3D cross-linked structure via boric acid-mediated hydrogen bonding and electrostatic interactions. The resulting BNLC-850 exhibited an impressive specific surface area of 1,822 m2 g-1, a well-defined hierarchical porous structure, and significant heteroatom content (12.65 at.% N, 5.67 at.% B, and 13.84 at.% O) (Figure 6j). The CV curves of the BNLC-850 electrode exhibit distinct humps, attributed to the Faradaic reactivity of B/N/O doping groups (Figure 6k). Among these doping functionalities, 89.68% of the pseudocapacitive sites are capable of directly participating in redox reactions. When used as an electrode, BNLC-850 showed a capacitance contribution of 65.31% (solid pattern) at 1 mV s-1 (Figure 6l,m), and achieved an impressive specific capacitance of 525.2 F g-1 (351.9 F cm-3) at 0.5 A g-1 in KOH. DFT calculations confirm that ternary doping reduces adsorption barriers for K-ions. Additionally, supercapacitors based on BNLC-850 demonstrate high energy density (41.2 Wh kg-1) and robust performance even at -20 °C. Table 2 summarizes previous research on the performance of carbon electrodes doped with different heteroatoms. High-content and multi-heteroatom doping can significantly enhance specific capacitance and energy density by introducing pseudocapacitance. However, excessive doping may increase ion diffusion resistance and induce electrochemical polarization, potentially compromising rate performance. Additionally, heteroatom doping appears to affect cycling stability, with doped carbons exhibiting around 90% stability after 10,000 cycles, compared to over 95% for pure carbon electrodes.
Samples | Surface area/m2 g-1 (pore volume/cm3 g-1) | Pore size (nm) | Heteroatoms doping (at.%) | Electrolyte | Capacitances/F g-1 (at current density/A g-1)(a) | Rate% (current density/A g-1)(a) | Energy density (Wh kg-1)(b) | Power density (kW kg-1)(b) | Device stability% (cycles) | Ref. |
N-doped carbon nanofiber | 618 (0.67) | 0.5-100 | N (10.63) | 1 M H2SO4 | 589 (0.5) | 52 (0.5-128) | 18.3-8.44 | 0.25-16.9 | 109.7 (90,000) | [13] |
P-doped carbon nanobowls | 1,343 (1.35) | 0.5-100 | P (1.8) | 6 M KOH | 273.6 (1) | 73.9 (1-20) | 7.12-5.9 | 0.5-4.3 | 94.9 (40,000) | [54] |
S-doped activated carbon | - | - | S (8.8) | 1 M H2SO4 | 440 (1) | 56.8 (1-10) | 18.14-9.75 | 0.89-9.99 | 90 (5,000) | [62] |
N/O enriched porous carbons | 761 (0.39) | 0.7, 2-50 | N (3.02) O (16.65) | 1 M H2SO4 | 370 (0.5) | 80.8 (0.5-30) | 10.3 | 0.12 | 92 (10,000) | [44] |
B/N doped 3D porous carbon | 641 (-) | 2.28 | N (7.8)) B (2.19) | 1M H2SO4 | 412 (1) | 69 (1-20) | 18.47 | 0.3 | 97 (10,000) | [55] |
N/S doped carbon spheres | 733 (0.65) | 1.6-3.7 | N (16.59) S (0.06) | 6 M KOH | 230 (0.5) | 74 (0.5-20) | 8.3 | 0.1 | 94 (5,000) | [63] |
B/N/Fe-doped carbon | 722 (-) | 0.2-20 | N (3.2) B (7.6) Fe (1.9) | 6 M KOH | 306 (0.5) | 81.6 (0.5-10) | 8.2 | 2.5 | 89.7 (5,000) | [64] |
B/N/P-doped carbon | 1,118 (-) | 1.0 | N (1.1) B (11.5) P (0.8) | 1 M H2SO4 | 518 (0.5) | 50.7 (0.5-100) | 12 | 0.7 | 91.6 (5,000) | [65] |
N doped micropore-rich carbon | 1,293 (0.71) | 0.7, 1.3 | N (20.87) | 6 M KOH | 400 (1) | 65 (1-20) | 18 | 0.325 | 97 (10,000) | [87] |
N/P/O-doped carbon spheres | 1,155 (-) | 2.5-60 | N (11.4) P (3.5) O (6.7) | 6 M KOH | 232 (0.5) | 68.1 (0.5-10) | 7.84-5.94 | 0.6-3.0 | 89.1 (5,000) | [91] |
N/P doped carbon nanosheets | 2,073 (1.35) | 0.5-15 | N (4.1) P (2.1) | 2 M KOH | 318 (1) | 59.8 (1-100) | 41.5-11.2 (NPCN/MnO2 as positive electrode) | 0.18-7.26 (NPCN/MnO2 as positive electrode) | 93.2 (10,000) | [94] |
N/B/O-enriched hierarchical porous carbons | 1,822 (1.50) | 2-100 | N (12.65) B (5.67) O (13.84) | 6 M KOH | 525.2 (0.5) | 67.7 (0.5-50) | 14.72-10 | 0.10-49.6 | 92.1 (10,000) | [18] |
(a): Specific capacitance and rate performance listed were obtained from three-electrode system; (b): E and P values listed were obtained from symmetric supercapacitors based on the carbon materials mentioned in the first column.
4.3 Engineering nanoarchitecture
4.3.1 0D carbon materials
Carbon/graphene quantum dots are a family of 0D carbon-based nanoparticles (< 10 nm). They typically feature a nanocrystalline core surrounded by an amorphous shell, which is rich in surface functional groups[96,97]. The choice of precursor is crucial as it influences the size, shape, and surface functionality of the resulting carbon-/graphene quantum dots. Common precursors include graphite, graphene oxide, and a variety of carbon-rich compounds such as CNTs, organic molecules, and biomass materials, have been used to prepare carbon/graphene quantum dots[98,68]. Highly crystalline GQDs, characterized by a rich edge position and a highly crystalline core are more suitable for supercapacitor electrode[67,92]. Zhang et al. prepared GQDs with a size of 1-3 nm through a top-down strategy[99]. The GQDs-based electrode demonstrated a specific capacitance of 297 F g-1 at 1 A g-1 and an impressive energy density of 22.2 Wh kg-1 at a power density of 10 kW kg-1. However, when bare quantum dots are used as electrode materials, their charge transfer efficiency between particles is relatively low, and they are prone to detaching from the current collector surface. Given the high-capacity performance enabled by the edge positions and surface functional groups of quantum dots, increasing research efforts have focused on anchoring carbon quantum dots or GQDs into conductive carbon matrices. This approach aims to develop high-performance composite materials or innovate new carbon-based structures. These structures utilize the unique properties of quantum dots, such as their large surface area, abundant functional groups, and quantum effects, to enhance the performance and versatility of carbon-based materials. Notable examples include hierarchical porous carbons, graphitized carbon frameworks, and composite materials with improved conductivity, ion transport, and surface reactivity. As depicted in Figure 7a, Wei et al. developed a hierarchical porous carbon material through the pyrolysis of a composite consisting of N, P co-doped carbon and GQDs embedded within a polyacrylamide hydrogel framework[100]. Carbon quantum dots with sizes of 5-8 nm can be found on the carbon skeleton (Figure 7b), revealing that the P and N groups originating from the carbon quantum dot precursors effectively adjust the electronic structure, generating electron-rich regions on the electrode surface. Its tunable surface chemistry and physical properties facilitate the adsorption of abundant cations without compromising the density and conductivity of the electrode. Consequently, an accelerated charge transfer process and a significant enhancement in the capacitance was achieved. The optimized material exhibited an outstanding specific capacitance of 468 F g-1 in 3 M KOH, 510 F g-1 in 4 M H2SO4, and 438 F g-1 in 1 M Li2SO4 electrolytes (Figure 7c), representing an increase of up to 70% compared to the sample in the absence of carbon dots. The crystallinity of carbon dots is important in enhancing ion and electron transport. The carbon quantum dots/hierarchical porous carbon composite maintains a retention rate of more than 80% at 30 A g-1 (Figure 7d).
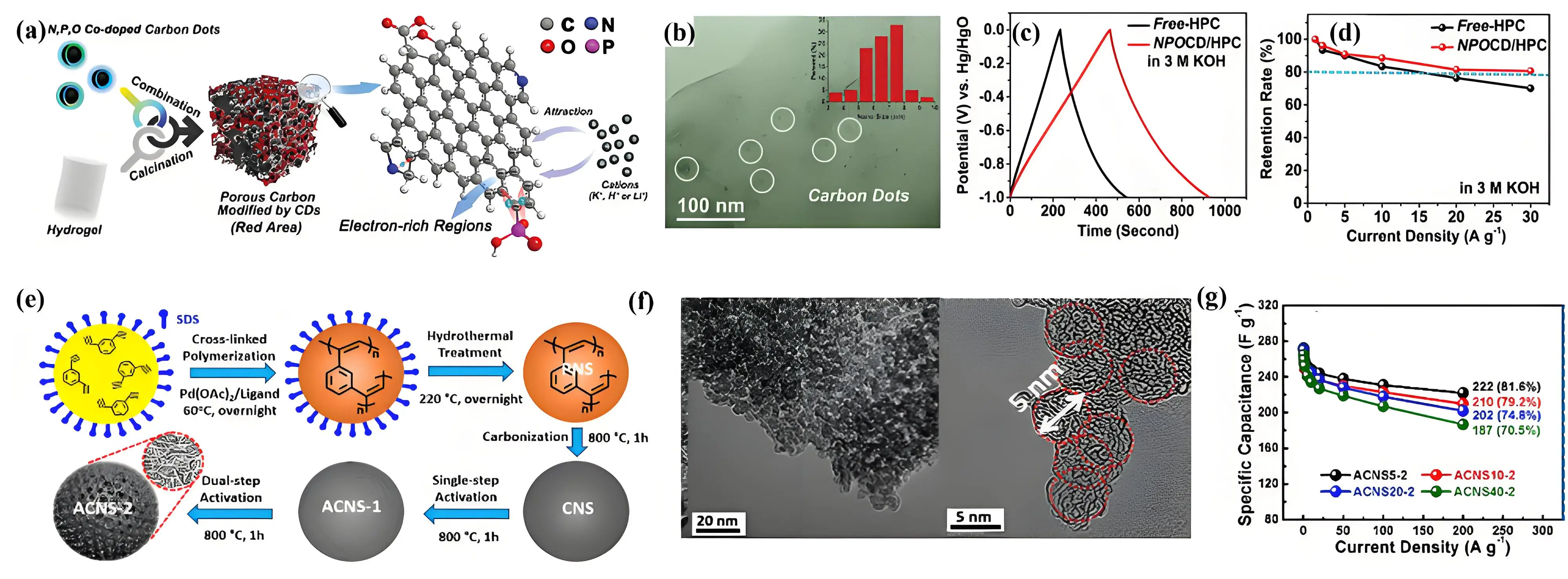
Figure 7. (a) Porous carbon derived from carbon dots and polyacrylamide hydrogels; (b) high-resolution TEM images and size distribution of carbon dots (inset); (c) GCD curves and (d) rate performance of porous carbon. Republished with permission from[100]; (e) synthesis mechanism; (f) TEM images; (g) rate performance of ultra-small CSs. Republished with permission from[47]. TEM: transmission electron microscopy; GCD: galvanostatic charge-discharge.
The creation of hierarchical porous carbon networks from carbon nanodots/GQDs with high conductivity and mechanical stability makes them suitable for electrochemical energy storage. Strauss et al. developed a laser-based technique to transform carbon nanodots into 3D turbostratic graphene. This process involved the thermolysis of citric acid and urea, followed by infrared laser irradiation[101]. The obtained 3D turbostratic graphene had a high active surface area of 230 m2 g-1 and a conductivity of 213 S cm-1 at optimal laser power. The hierarchical porosity, excellent electrical conductivity, and fast charge-discharge behavior favors an improved specific volumetric capacitance of 27.5 mF dm-3 at 560 A dm-3, along with a high energy density of 24.1 mWh L-1 (17.7 Wh kg-1) at a power density of 711 W L-1 (2.55 kW kg-1). Tian et al. developed a conductive porous carbon material using crystalline GQDs as the precursor and a block copolymer as a soft template through a micelle-induced assembly method[102]. The resulting material featured an interconnected mesoporous structure with high specific surface area (1,323 m2 g-1) and impressive conductivity (73 S m-1), which promoted efficient electron and ion transport even under high mass loading conditions. Performance testing revealed an outstanding capacitance of 315 F g-1 at 1 A g-1 in 6M KOH, with excellent rate capability, retaining 67.3% and 54% of its capacitance at 50 A g-1 and 100 A g-1, respectively.
Porous carbon nanospheres are another type of 0D carbon material. Carbon nanospheres exhibit inherent advantages in rapid charge transport and storage due to their adjustable pore size distribution, tunable shell layers, and unique surface properties[103,104]. Designing the structures of carbon nanospheres is crucial to enhance their electrochemical performance. The nanospheres can be prepared through the direct carbonization of polymer spheres and hydrothermal carbonization of carbon precursors. For example, Xue et al. synthesized N and O-rich carbon nanospheres by direct carbonization/activation of melamine-glyoxal polymers[105]. Due to their well-developed micro- and mesoporous structures, the carbon spheres achieved a specific capacitance of 344 F g-1 at 1 A g-1. However, their larger size (800 nm) increases the ion diffusion distance within the pores, resulting in limited capacitance retention (62%) from 1 to 20 A g-1. In contrast, smaller carbon spheres with optimized pore structures provide better accessibility for the electrolyte solution to reach internal pores, reducing both mass transfer and charge transfer resistance. This enhancement effectively improves capacitance and rate performance[106]. N-doped carbon nanospheres, with well-ordered mesoporous channels and a uniform size of 300 nm, demonstrate impressive performance by maintaining 66% of their capacitance even at a high current density of 50 A g-1[107]. Liu et al. synthesized carbon nanospheres with controlled diameters ranging from 5 to 40 nm using an emulsion polymerization method (Figure 7e,f), followed by a dual-step KOH activation[47]. The ultra-small size and microporous/mesoporous structure of the spheres contributed to their excellent high-rate performance, achieving capacitance retention rates of 70.5-81.6% (Figure 7g). Reducing the nanosphere size from 40 nm to 5 nm significantly improved rate performance by enhancing ion transport within the spheres. The optimized carbon nanospheres, with an average size of 5 nm, demonstrated exceptional performance, achieving a specific capacitance of 272 F g-1 at 0.5 A g-1 and retaining 81.6% of their capacitance even at a high current density of 200 A g-1 in 1M H2SO4.
In addition to solid carbon nanospheres, hollow-structured carbon nanospheres offer a high surface-to-volume ratio that shortens mass and charge transport paths. This structure also effectively mitigates the volume expansion of electrode materials during the charge-discharge process, resulting in high capacitance retention and an extended cycle life. Hollow carbon spheres are usually prepared using the template method. This process entails applying a carbon precursor onto a template surface, which is then subjected to high-temperature carbonization[108,109]. In contrast, soft templates (such as nano-emulsion droplets, micelles, and MOFs) can be removed more easily through high-temperature pyrolysis[63,110]. For hollow carbon spheres, parameters such as shell thickness, surface roughness, pore size within the shell, and spatial structure play a critical role in influencing electrolyte transport. Zhang et al. synthesized hollow N-doped carbon spheres through high-temperature pyrolysis of hollow zinc-based metal-organic framework spheres[17]. These spheres exhibited a specific capacitance of 254 F g-1 at 1 A g-1 in 6M KOH and retained 99.9% of their capacity after 10,000 cycles. However, the microporous shell structure limited ion transport, resulting in only 61.3% capacitance retention at 10 A g-1. To address this, Zhang et al. introduced hierarchical meso-macroporous structures into the shell of hollow carbon spheres[90]. The microporous-mesoporous-macroporous shell structure significantly enhanced the surface's electrochemical activity, facilitated ion and electron transport, and improved capacitance retention. At current densities of 10 A g-1 and 20 A g-1, the specific capacitance retained 81% and 76% of its value at 0.5 A g-1, respectively. Additionally, it has been shown that rate performance declines as shell thickness increases[111]. A thinner shell improves the rate performance of hollow carbon spheres. The capacitance retentions of the thinnest shell samples reach 84.1% (0.5-10 A g-1), approximately 25% higher than that of thicker-shell hollow carbon spheres. Overall, carbon spheres with small sizes, hollow architectures, and hierarchical porous structures can effectively promote ion storage and electron transport, enhancing electrochemical activity and exhibiting high capacitive performance, fast ion diffusion rate, and high stability.
4.3.2 1D
Although 0D materials offer a high specific surface area and a tunable structure, their lack of a continuous conductive network often leads to low electrical conductivity, restricting their efficiency in electron transfer. In contrast, the unique advantages of 1D nanostructured carbon electrodes lie in their ability to provide a continuous charge transport network and reduce contact resistance, which is crucial for high-power-density supercapacitors.
4.3.2.1 CNTs
With their covalent sp2 bonds, CNTs provide exceptional mechanical strength, thermal stability, and electrical conductivity, making them highly valuable for energy storage applications. The specific capacitance of CNTs depends on their purity and morphology. High-purity CNTs (free of catalyst residues and amorphous carbon) typically have a specific surface area of 120-400 m2 g-1 and a specific capacitance of 15-80 F g-1[112,113]. To enhance the specific capacitance, CNTs can be activated with KOH to introduce a large number of micropores while retaining the nanotube structure. This activation increases the specific surface area to around 1,035 m2 g-1[114]. Oxidation treatment with acids (e.g. sulfuric acid, nitric acid) can also be used to modify the surface morphology of CNTs and introduce oxygen-containing functional groups, providing additional pseudocapacitance. However, excessive activation can deteriorate their electrical conductivity. CNTs can grow directly on substrates without the need for additional binders, reducing the resistance between the active material and the current collector while significantly simplifying electrode preparation. However, the specific capacitance of pure CNTs as supercapacitor electrode materials remains relatively low. Furthermore, compared to traditional electrode materials like activated carbons, the stringent synthesis conditions, complex preparation methods, and high cost of CNTs make them difficult to commercialize[115,116]. Therefore, CNTs are often used as conductive substrates or structural additives in flexible supercapacitors owing to their excellent electrical conductivity and outstanding mechanical properties[117].
4.3.2.2 Carbon nanofibers
Carbon nanofibers are capable of integrating high performance and diverse functionalities. Electrospinning is a widely used and effective method for fabricating carbon micro/nanofibers[118]. Polyacrylonitrile is extensively employed as a precursor for electrospun carbon nanofibers[119]. Pure polyacrylonitrile-based carbon nanofibers exhibit a dense structure, resulting in limited ion diffusion pathways and a significantly reduced accessible surface area[120]. As a result, the specific capacitance is only 173 F g-1 at 10 mA g-1. The specific capacitance of carbon nanofibers can be enhanced through the introduction of porous structures, heteroatom doping, and surface modification with active materials[121]. Various sacrificial templates, such as polymers[122,123], SiO2 spheres[124], and polyacrylonitrile-containing copolymers[125,126], have been employed to fabricate porous carbon nanofibers. Each sacrificial material imparts distinct pore structures and properties to the resulting carbon nanofibers. For instance, incorporating poly(methyl methacrylate) creates hollow structures and promotes the formation of mesopores. Poly(acrylonitrile-block-methyl methacrylate) facilitates microphase separation, producing porous carbon nanofibers with interconnected micro-/mesoporous structures. Meanwhile, the addition of polystyrene results in carbon nanofibers with independent nanochannels aligned along the fiber axis. The porosity can be also enhanced through activation treatments. Different physical or chemical activation processes, such as those using steam, CO2, or KOH/NaOH, can be applied to create pores within the carbon nanofibers[121,127]. For instance, porous hollow carbon nanofibers can be fabricated by activating electrospinning carbon nanofibers with NaOH[128]. The sample carbonized at 800 °C achieved a specific surface area of 2,628 m2 g-1 and a maximum pore volume of 2.32 cm3 g-1. Electrochemical tests revealed a high specific capacitance of 330 F g-1 at 1 A g-1 with 65.3% capacitance retention at 20 A g-1. However, the resulting pores are predominantly micropores with diameters smaller than 2 nm, making it challenging to precisely control pore size distribution and overall porosity in the carbon nanofibers.
Engineering the internal and external porous structure is an efficient approach to improving the electrochemical performance of carbon nanofibers. For instance, incorporating GQDs into electrospun carbon nanofibers significantly enhances their internal porous structure[129]. The uniformly dispersed GQDs fulfill a dual function by reinforcing the structural framework and establishing a conductive network[130]. During the preoxidation phase, the polyacrylonitrile matrix in precursor fibers exhibits reactive hydrogen atoms on its molecular backbone. At elevated temperatures, these hydrogen atoms react with -OH groups on the GQDs through dehydrogenation or dehydration processes. Consequently, GQDs act as cross-linking agents, bridging the molecular chains of polyacrylonitrile and fostering the development of a dense, sturdy carbon framework (Figure 8a). Additionally, GQDs reduce the crystalline domain size within carbon nanofibers by introducing extra nucleation sites, which promote grain refinement. The GQDs in carbon nanofibers have a lattice spacing of 0.242 nm, corresponding to the (1100) crystal plane of graphite (Figure 8b). Compared to pristine carbon nanofibers, the GQD-activated carbon nanofibers show a remarkable surface area expansion from 140 m2 g-1 to 2,032 m2 g-1, along with notable improvements in conductivity and mechanical strength, which increase by 5.5-fold and 2.5-fold, respectively. As an independent supercapacitor electrode, it delivers a specific capacitance of 335 F g-1 at 1 A g-1 (Figure 8c), maintaining a capacitance retention of 77% at 100 A g-1 and 45% at 500 A g-1 (Figure 8d). Remarkably, the symmetric device charges to 80% of its capacity in just 2.2 seconds, highlighting its potential for high-power start-up applications. The external porous structure can also be designed to improve the capacitance[131,132]. Kim et al. grew highly porous N-doped CNTs on hollow carbon nanofibers through a metal–organic framework-assisted thermal treatment method (Figure 8e)[131]. The N-doped CNTs, with lengths of 3μm and diameters ranging from 5 to 20 nm densely cover the hollow carbon nanofibers (Figure 8f,g). The cross-sectional morphology reveals the hollow carbon nanofibers core (highlighted by the yellow cursor) and the nanofibril sheath (indicated by the red cursor). Transmission electron microscopy (TEM) images display a consistent cluster of highly porous CNTs, with Co nanoparticles, both single and aggregated, firmly embedded within the CNTs (Figure 8h,i). The optimized carbon nanofibers achieve an exceptional capacitance of 712 F g-1 at 1A g-1 in an aqueous electrolyte, along with a rate performance maintaining 67.7% at 30 A g-1.
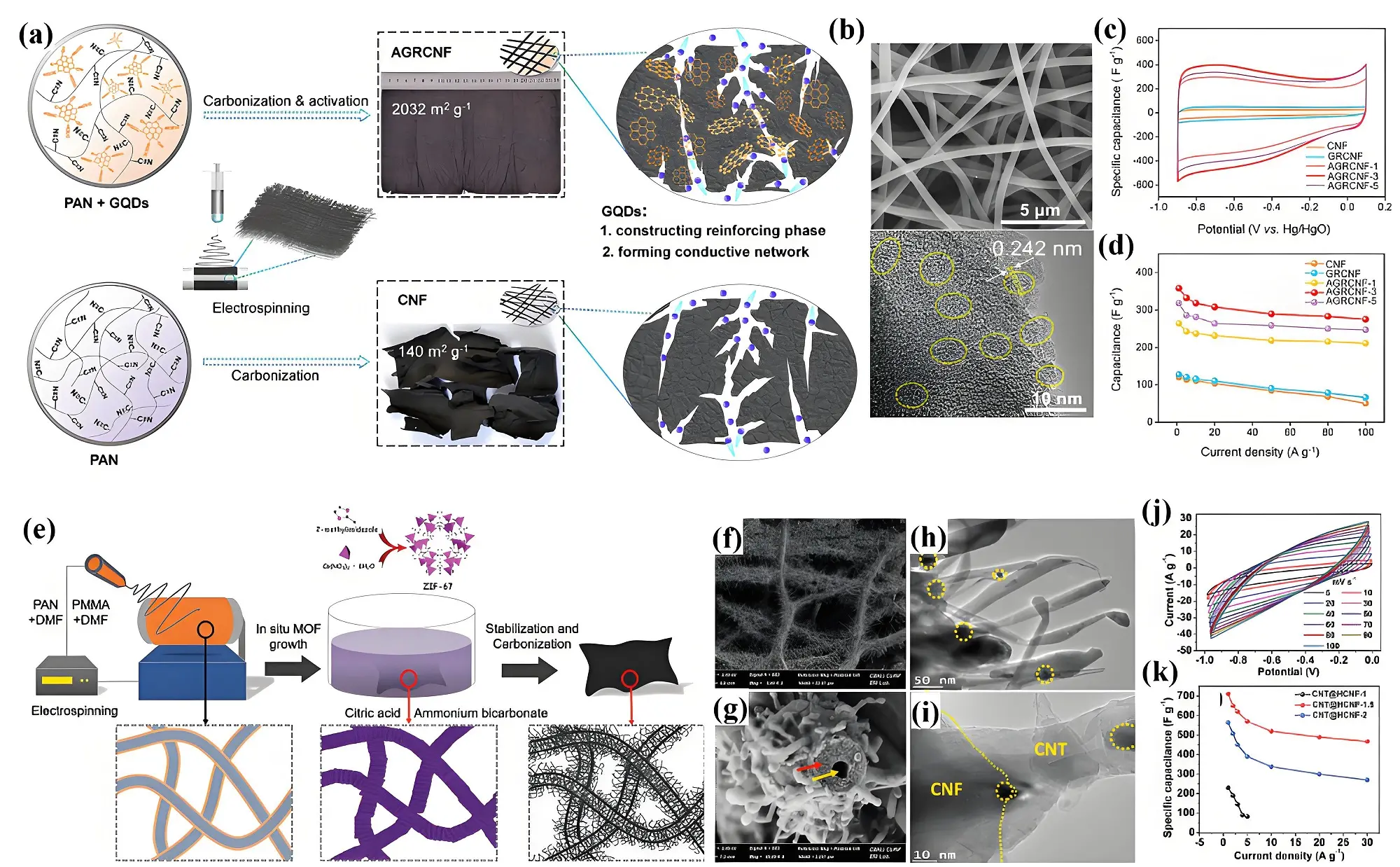
Figure 8. (a) Formation mechanism; (b) SEM and TEM images; (c) CV curves; (d) rate performances of GQDs modified carbon nanofibers. Republished with permission from[130]; (e) formation mechanism; (f-i) SEM and TEM images; (j) CV curves; (k) rate performances of CNTs modified carbon nanofibers[131]. SEM: scanning electron microscope; TEM: transmission electron microscopy; CV: cyclic voltammetry; GQDs: graphene quantum dots; CNTs: carbon nanotubes; PAN: polyacrylonitrile; AGRCNF: activated graphene quantum dots-embedded carbon nanofiber fabric; CNF: carbon nanofiber; DMF: dimethyl formamide; PMMA: poly(methyl methacrylate); MOF: metal-organic framework.
Graphene, with its unique sp2-hybridized two-dimensional planar structure, lightweight, exceptional strength (around 130 GPa), and the highest electrical conductivity among known carbon materials due to its conjugated π-bond system, is an ideal material for producing high-performance fibers. The graphene fibers are primarily derived from graphene oxide, which is soluble in organic solvents. These graphene oxide fibers are then transformed into graphene fibers through suitable reduction techniques (e.g. chemical reduction or high-temperature reduction). Gao et al. first prepared a liquid crystalline solution of graphene oxide and fabricated graphene fibers using a wet-spinning method. The resulting fibers exhibited excellent tensile strength (140 MPa) and electrical conductivity (2.5 × 104 S m-1[133]. Their mechanical properties (tensile strength up to 1.78 ± 0.15 GPa) and electrical conductivity (8 × 105 S m-1) can be significantly enhanced through various approaches, such as increasing the size of graphene oxide sheets, employing different coagulation baths, high-temperature post-treatments, and doping[59,134-136]. While these strategies promote graphitization within the fibers, enhancing their mechanical strength, modulus, and conductivity, they often come at a cost. Graphene fibers with high conductivity frequently lose their porous structure, sometimes becoming more solidified. This transformation limits electrolyte access to the outer surface of the electrode, drastically reducing the accessible surface area and ion diffusion rates, which severely impairs their charge storage capacity and rate performance. To address this issue, improvements to graphene fibers have focused on increasing the specific surface area of the outer surface and enhancing the internal pore structure[17,59]. For example, fabricating graphene fiber electrodes with a hollow structure creates additional surface area within the interior, enhancing electrolyte contact and significantly boosting their capacitive performance[137]. Chen et al. developed a scalable non-liquid crystalline spinning process[138]. As illustrated in Figure 9a, adjusting the pH to 11 with NaOH disrupts the hydrogen-bonding network in the pristine liquid crystal phase, enhancing the flowability of the non-liquid crystalline graphene oxide (GO) dispersion. The resulting fibers exhibit a bark-like surface microstructure, cross-sections with varied shapes, and densely packed pores localized in specific regions (Figure 9b). By tuning the stretching ratio during the spinning process, the fibers achieve a conductivity range of 815-1,530 S m-1. Loosely stacked graphene fibers exhibit numerous wrinkles on both their internal and external surfaces. These fibers achieve a specific capacitance of 279 F g-1 (340 F cm-3) at 0.2 A g-1 (0.244 A cm-1) in 1 M H2SO4. Supercapacitors based on these graphene fibers deliver a volumetric capacitance of 266 F cm-1 and an energy density of 7.03 mWh cm-3 at 0.244 A cm-3. Leveraging the internal interfaces of the fibers to enhance electrochemical activity is crucial. The fibers feature a hierarchical porous structure with pore sizes ranging from sub-nanometer to over 100 nanometers, providing efficient ion-accessible pathways for rapid charge transfer. Qiu et al. proposed a straightforward magnetothermal microfluidic approach for the rapid expansion of internal porous framework of graphene fiber to enhance ionic diffusion at the electrode/electrolyte interface and throughout the fiber's interior[139]. As shown in Figure 9c, graphene oxide and hydrogen peroxide were injected into a T-shaped channel and mixed under intense ultrasonic treatment to form a well-dispersed solution. The dispersion was then directed into a stainless-steel tube. The induction coil generated a magnetic field that rapidly heated the tube, reaching 90 °C in just a few seconds. This rapid heating triggers an immediate reaction in the mixed dispersion, resulting in the formation of nanoporous structure. Meanwhile, carbon atoms in the active defect regions of graphene oxide were oxidized and etched by H2O2, gradually forming nanopores. The resulting graphene fiber features an interconnected hierarchical porous structure, with micro- to nanoscale pores distributed across both its outer surface and interior (Figure 9d). It also demonstrated outstanding conductivity (13,290 S m-1) and a significantly higher specific surface area of 569 m2 g-1, compared to pure graphene fiber (173 m2 g-1). This led to an impressive capacitance of 2,760 mF cm-2 at 0.1 mA cm-2. Urea was used as both a nitrogen source and a template to fabricate N-doped graphene fiber electrodes with hierarchical micro-, meso-, and macropores[140]. Increasing the urea content further expanded smaller pores into macropores, creating a more loosely stacked graphene sheet structure. As a result, the supercapacitors delivered a high areal capacitance of 1,217 mF cm-2 at 0.1 mA cm-2 and an energy density of 27 μWh cm-2 at a power density of 40 mW cm-2 in polyvinyl alcohol/H3PO4 gel electrolyte.
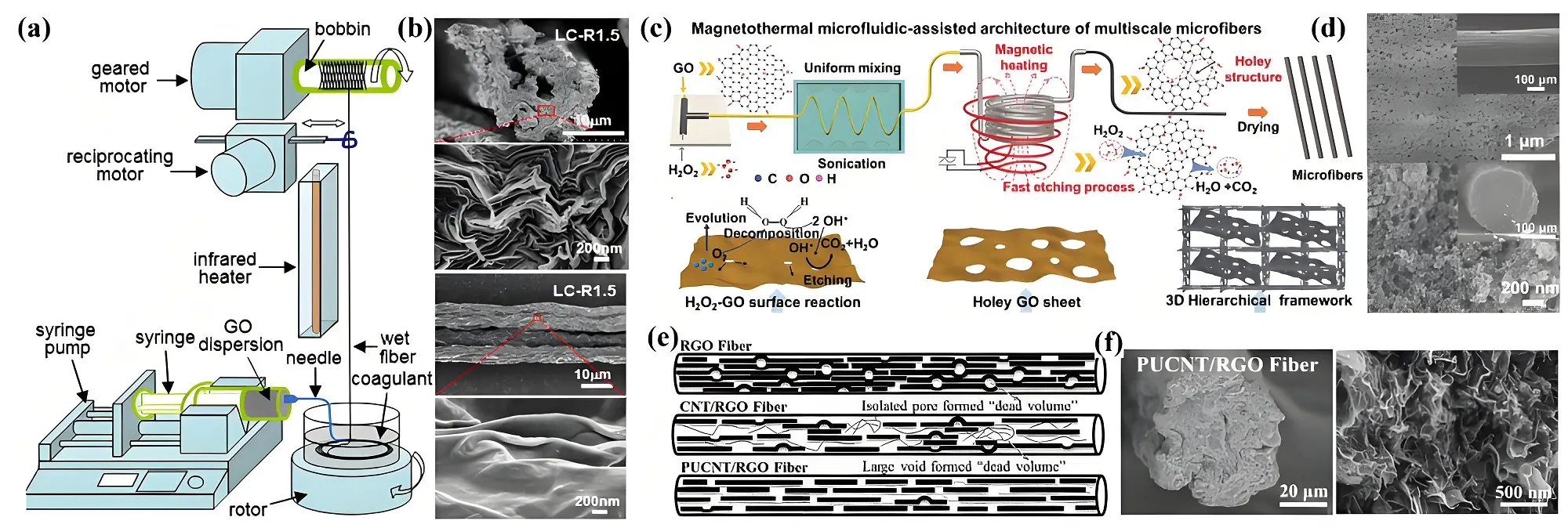
Figure 9. (a) Non-liquid crystal wet spinning process; (b) SEM images of graphene fibers[138]; (c) microfluidic synthesis process to prepare; (d) SEM images of hierarchical porous graphene fiber. Republished with permission from[139]; (e) structures graphene fibers using partially unzipped carbon nanotube as spacer; (f) SEM images of partially unzipped oxidized carbon nanotube/reduced graphene fiber. Republished with permission from[142]. SEM: scanning electron microscope; GO: graphene oxide; PUCNT: partially unzipped carbon nanotube; RGO: reduced graphene oxide.
In addition, it has been demonstrated that when graphene consists of single or a few layers, the capacitance exhibits an unusual increase, resembling battery-like behavior. This anomaly is likely attributed to the interaction between the p-band Fermi liquid and the ions in the Helmholtz layer. As the number of graphene layers increases, capacitance decreases due to electrostatic shielding[22]. To address this, various spacers have been introduced within graphene fibers to expand the interlayer spacing and enhance charge storage capacity[141]. Partially unzipped carbon nanotubes have been utilized as spacers to prevent the restacking of graphene oxide sheets (Figure 9e). The resulting reduced graphene oxide hybrid fiber minimizes "dead volume" and exhibits a uniform circular cross-section with a looser structure (Figure 9f). The study also revealed that using a high-concentration spinning solution improves mass transfer, effectively reducing the formation of solely pores[142]. Carbon dots were also served as reinforcing nanofillers to prevent the restacking of graphene sheets through a microfluidic technique[143]. The strong interaction between carbon dots and graphene leads to a dot-sheet structured fiber. The fabricated graphene fibers exhibit a wrinkled surface and a porous network, with carbon dots uniformly embedded within the graphene nanosheets. This dot-sheet structure creates abundant micro- and mesopores, significantly enhancing ion transport and charge storage. The resulting graphene fibers achieve a specific surface area of 435.1 m2 g-1, which is 1.8 times higher than that of pure reduced graphene oxide fibers, and deliver a capacitance of 607 mF cm-2 at 0.1 A cm-2 in 1 M H2SO4, approximately three times greater than that of pure reduced graphene oxide fibers. This performance improvement is largely attributed to the contribution of the carbon dots, which account for 22.1% of the total capacitance by facilitating hydrogen bonding and dehydration-condensation interactions, thereby enhancing ion diffusion and charge accumulation.
4.3.3 2D carbon materials
Unlike 1D carbon materials, whose surface area is highly dependent on their porous structure, 2D graphene/carbon sheets with layered porous architectures offer a distinct advantage. Their large surface area is independent of pore structure, while their unique architecture facilitates efficient electrolyte penetration and ion accessibility by minimizing ion transport resistance and shortening diffusion distances. This makes them a promising solution for optimizing the balance between surface area and electrical conductivity in carbon materials. Additionally, their high conductivity ensures low-resistance pathways for electron transport, making them a compelling candidate for enhancing the performance of carbon-based electrodes[144].
Biomass-derived carbon materials offer a unique advantage due to their naturally occurring hierarchical structures. These structures can be preserved or further refined during pyrolysis to produce carbon nanosheets with high surface area and tailored pore architectures. When heat-treated, especially in the presence of metal catalysts, the carbon in biomass undergoes graphitization and bond rearrangement, resulting in the formation of graphene-like nanosheets. Metal catalysts play a crucial role in breaking and reorganizing carbon bonds into the desired sheet-like structures. Additionally, chemical activating agents like ZnCl2 or KOH can be introduced during the pyrolysis process to create abundant pores and exfoliate the biomass into thin, nanosheet-like carbon materials[145-147]. Lian et al. developed ultrathin carbon nanosheets with a controllable thickness using an ice-templated natural biopolysaccharide gel as a precursor[64]. Through selective co-doping with elements such as Fe, B, and N, structural defects were introduced into the carbon lattice to enhance the material's performance. B doping was found to preferentially convert pyrrolic nitrogen into B-N sites, which possess lower energy barriers for adsorption, thereby improving the capacitive properties of B, N co-doped carbon. Additionally, the electronic interaction between N and B optimized the material's conductivity, accelerating charge transfer kinetics. Iron acted as both an activator and a graphitization catalyst, enhancing the degree of graphitization while creating a rich microporous structure on the nanosheet surface. These combined effects significantly increased the specific surface area and electrical conductivity. The resulting Fe-CNB material achieved a capacitance of 306 F g-1 at 0.5 A g-1 and maintaining a high rate performance of 79% at 10 A g-1.
As mentioned earlier, 2D graphene offers benefits like excellent electrical and thermal conductivity, along with impressive mechanical strength. However, the strong van der Waals forces and π-π interactions cause reduced graphene oxide nanosheets to stack, which limits the effective use of their specific surface area and negatively affects performance[148]. Functionalization, pore introduction, or structural modifications to graphene layers can significantly improve the energy storage performance of 2D graphene materials[22,149]. Graphene films are typically prepared from a graphene oxide dispersion, followed by various film processing techniques such as vacuum filtration, mechanical pressing, screen printing, or solvent evaporation-induced self-assembly. The interlayer spacing, thickness, mechanical strength and electrical conductivity of the resulting films can be effectively tailored by modifying the precursor suspension[150]. However, due to the scrolling of monolayer graphene oxide and the decomposition of oxygen functionalities during the reduction process, the resulting graphene often forms some isolated pores, which is not useful for charge storage. By integrating the merits of graphite, graphene, and graphene oxide, Fu et al. developed surface-oxidized multilayer graphene through electrochemical exfoliation of graphite papers in H2O2 and (NH4)2SO4 solution[151]. The multilayer graphene primarily consists of 4-5 layers with oxidized surface (oxygen content of 10%), while the interior remains largely unoxidized. The graphene film combines high density, excellent charge transfer capabilities, and oxygen-active sites, achieving a capacitance of 164 F cm-3 at 0.85 A cm-3 in 3M KOH.
Introducing spacers between graphene layers is an effective way to mitigate stacking and aggregation. However, the compact and continuous structure of graphene sheets significantly limits the vertical transport of electrolyte ions. To overcome this, creating in-plane pores can enhance ion diffusion and provide additional active reaction sites, making it an effective strategy for enhancing the performance of graphene-based supercapacitors. The generation of pores in graphene/carbon sheets via liquid-phase oxidation or air oxidation has been widely reported[152-154]. Xu et al. developed holey carbon sheets through a pyrolysis-leaching process using potassium phthalimide as a precursor[19]. During pyrolysis, potassium salts form and serve as in situ activation agents, promoting the creation of highly porous carbon structures with a large surface area (Figure 10a). These holey carbon sheets exhibit a high specific surface area of 1,004 m2 g-1, featuring hierarchical pores and O (10.2 at%) and N (6.64 at%) dopants. Scanning electron microscope (SEM) and TEM images revealed the 2D nanosheet structure of the carbon nanosheets, along with the presence of numerous pores distributed across their surface (Figure 10b). These structural features enable fast ion transport and abundant active sites, resulting in exceptional performance (454 F g-1 at1 A g-1) even with thick electrodes and limited electrolyte. They also investigated the influence of mass-loading on the capacitance performance and found that when the mass elevated to seven times (2.40 to 16.1 mg cm-2), the charge storage performance only decreased by 11.5% (Figure 10c).
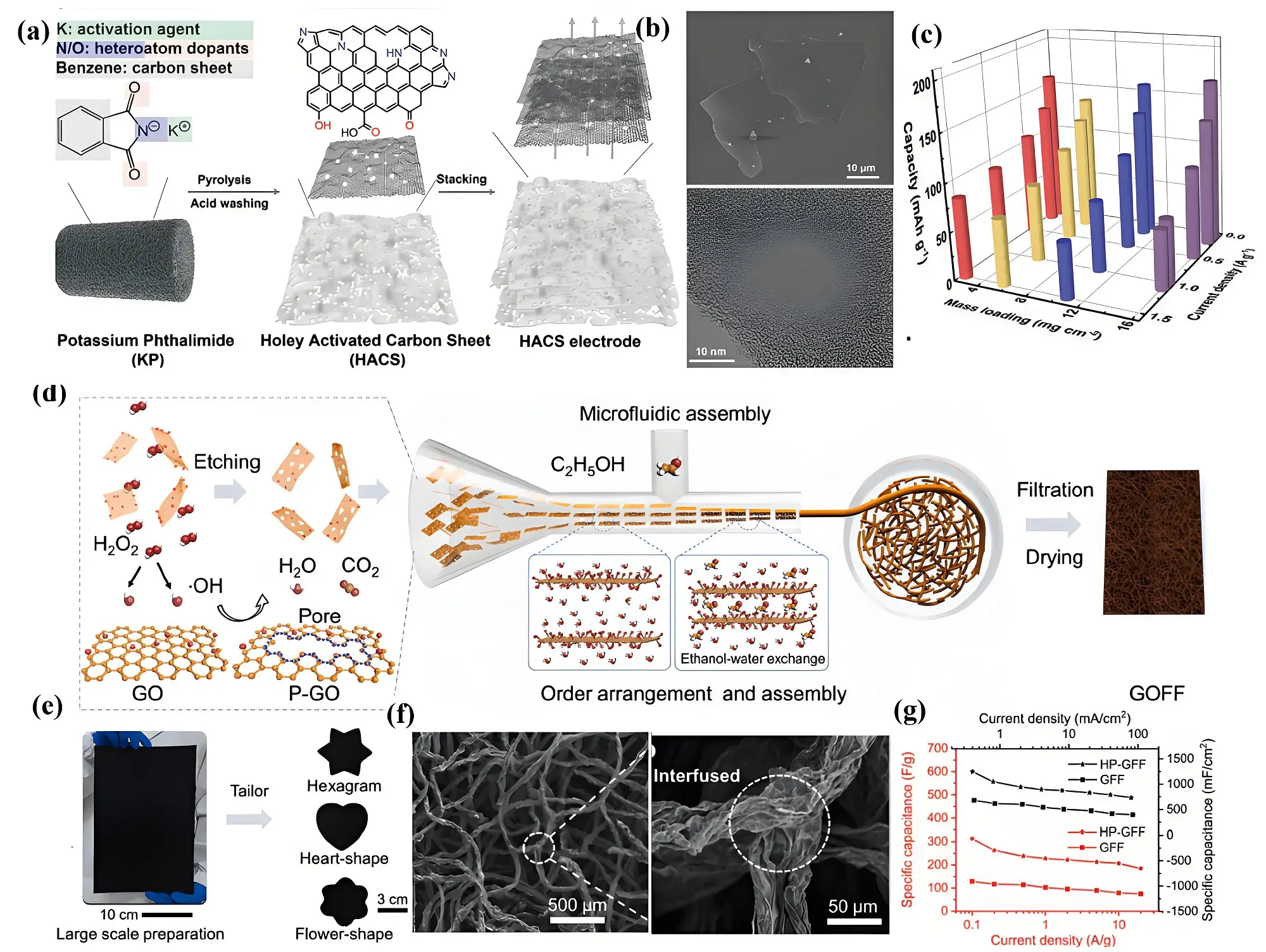
Figure 10. (a) Synthesis mechanism; (b) SEM and TEM images; (c) capacity of different mass loading of holey graphene sheets[19]; (d) schematic illustration; (e) different shaped GFF; (f) SEM images; (g) capacitance of H2O2 activated GFF[158]. SEM: scanning electron microscope; TEM: transmission electron microscopy; GFF: graphene fiber fabric; GO: graphene oxide; GOFF: graphene oxide fiber fabrics.
Building on the advantages of graphene-based materials, graphene fiber fabrics (GFFs), crafted from graphene fibers, have recently gained attention as a promising 2D material. Gao et al. pioneered a wet-fusing assembly technique to develop GFFs by intertwining graphene fibers, representing a significant advancement in the development of nonwoven graphene fiber materials[155]. They later introduced hydrothermally activated GFFs with improved porous architectures, tailored specifically for supercapacitor electrodes. A single 150 μm-thick graphene fiber fabric (GFF) layer demonstrated a specific capacitance of 1,060 mF cm-2 at 1 mA cm-2 (244 F g-1 at 0.1 A g-1) and high rate performance (84% at 100 mA cm-2) in 1M H2SO4[156]. However, stacking multiple layers to enhance areal capacitance posed a challenge, as it significantly reduced the rate performance. To address these limitations, Shao et al. incorporated hydrazine into the coagulation bath to enable cross-linking and reduction of graphene fibers, resulting in a GFF with a mass loading of 14.2 mg cm-2. This GFF achieved a capacitance of 2,812 mF cm-2 at 1 mA cm-2 (220 F g-1 at 1 A g-1) with a capacitance retention of 84% at 10 mA cm-2 in 1M H2SO4[157]. As depicted in Figure 10d, Guan et al. introduced a novel approach for further enhancing GFF properties through precise microfluidic assembly and H2O2 etching techniques, creating an open interconnected porous structure with a large accessible surface area of 221 m2 g-1[158]. This process enabled large-scale production (30 × 20 cm) and adaptability to various shapes (Figure 10e,f), driven by the formation of tightly interfused junctions at fiber nodes through capillary forces and hydrogen bonding interactions. The resulting GFF featured uniformly distributed graphene fibers, averaging 50μm in diameter, with a random in-plane alignment and noticeable wrinkles. These fibers were seamlessly fused at their intersections, forming strong and well-defined junctions that improved structural integrity and performance. The porous architecture and interconnected pathways of the GFF facilitated efficient migration and intercalation of electrolyte ions, leading to exceptional electrochemical performance. The hydrothermally processed GFF (HP-GFF) achieved a specific capacitance of 1,248.5 mF cm-2 at 0.1 mA cm-2 (312.9 F g-1 at 0.1 A g-1) and demonstrated rate performance of ~ 63% at 100 mA cm-2 in 1M H2SO4 electrolyte (Figure 10g). These advancements highlight the potential of GFFs as a flexible and scalable solution for high mass-loading and high-performance supercapacitor applications.
4.3.4 3D carbon materials
For advanced electrochemical applications, 3D carbon architectures stand out due to their interconnected porous networks and robust mechanical properties. These architectures are designed to promote efficient ion transport through their interconnected pores, enabling extensive interaction between the electrode and electrolyte. This structure not only enhances ionic accessibility and diffusion rates but also supports rapid charge transfer by improving electronic conductivity, positioning them as excellent options for high-performance electrode materials. Honeycomb-like hierarchical porous carbon is a typical 3D porous carbon[58]. Such materials can be fabricated through direct carbonization of some biomass materials with intrinsic porous structures, such as popcorn[159], petroleum asphalt[160,161] and chitosan[162]. Sun et al. developed N and O co-doped honeycomb-like carbon by crosslinking chitosan in the array channels of mantis shrimp shell[163]. The removal of the mantis shrimp shell, followed by KOH activation, facilitated the creation of numerous micropores (0.5-0.8 nm and 1.18-1.48 nm) and mesopores (~ 2.73 nm). The prepared hierarchical porous carbon exhibited a high surface area of 2,951 m2 g-1 and pore volume of 1.84 cm3 g-1. Based on these characteristics, the porous carbon achieved a specific capacitance of 401 F g-1 (0.5 A g-1) in 6 M KOH and a rate performance of 70.3 % at 20 A g-1, and a high energy density of 13.3 Wh kg-1 (145 W kg-1) in 6M KOH and 82.5 Wh kg-1 (437.5 W kg-1) in organic electrolyte. Amino acids were also utilized as structure-directing agents to crosslink chitosan, forming a honeycomb-like porous structure, while boric acid served as a reactive template to generate additional pores[164]. The resulting B/N co-doped porous carbons exhibited a high surface area of 2,362 m2 g-1, with dominant pore sizes below 2 nm and between 2 and 4 nm. These materials achieved a capacitance of 478 F g-1 at 0.5 A g-1, while maintaining 53.9% of their capacitance at 50 A g-1.
In addition, the direct carbonization of commercial melamine sponges or polyurethane sponges leads to the formation of lightweight, durable free-standing N-doped carbon sponges with interconnected networks[165-167]. Xu et al. developed a 3D hierarchical carbon material by integrating polyurethane sponge templating with KOH activation of GO platelets. In this process, the sponge template guided the alignment of GO platelets along its structure, while KOH activation generated a network of micropores and mesopores (0.48-4 nm) within the sponge during thermal annealing. This dual approach resulted in a highly porous carbon material with a surface area of 2,582 m² g-1 and excellent electrical conductivity (2.41 Ω sq-1). The 3D interconnected structure and internal porosity significantly shortened ion transport distances, leading to outstanding electrochemical performance, including a specific capacitance of 401 F g-1 at 5 A g-1 in 1.0 M H2SO4, with 87% retention at a current density of 100 A g-1[168]. It was found that the pore size could be tuned by varying the KOH-to-melamine sponge mass ratio. As the amount of KOH increased, both pore size and quantity expanded, with the proportion of mesopores and macroporesincreasing from 27% to 34%[169].
Freeze-drying is one of the most popular method for fabricating 3D carbon foam/aerogel[20]. As shown in Figure 11a, Peng et al. designed a carbon aerogel enriched with N, O, and S through a combination of soft templating, freeze-drying, and chemical etching[170]. The resulting carbon foam has an ultra-low density of just 20.9 mg cm-3 (Figure 11b) and exhibits a macroporous structure with interconnected mesopores and micropores, achieving an impressive specific surface area of 2,685 m2 g-1. SEM images (Figure 11c) illustrate its macroporous architecture, which is formed by wrinkled carbon nanosheets densely populated with mesopores (Figure 11d). The nanosheet thickness and mesopore size can be precisely controlled by varying the polymerization time. Notably, a longer polymerization time results in thicker nanosheets and smaller mesopores, with the pore size shrinking from 18.4 ± 4.4 nm to 8.8 ± 2.4 nm as the duration increases from 1 to 20 hours. Additionally, TEM images confirm that these mesopores are vertically aligned channels with an approximate diameter of 10 nm, providing further structural insight. The N,O,S-enriched carbon aerogel electrode demonstrates remarkable electrochemical performance, achieving 402.5 F g-1 at 1 A g-1 in 6 M KOH, along with high rate capability, maintaining 92.3% at 10 A g-1 and 76.6% at 100 A g-1. Considering the 3D hierarchical porous structure, which offer a larger electrochemical active surface area that reduces ion diffusion resistance and distance, Yao et al. focused on carbon aerogel-based supercapacitors used under ultra-low temperature[171]. 3D printing and cellulose nanocrystal-based ink have been employed to develop a multiscale porous carbon aerogel with an impressive surface area of 1,749.7 m2 g-1 (Figure 11f). The cellulose molecules, with six hydroxyl groups each, form strong hydrogen bonds both internally and with water, ensuring structural stability. To produce a uniform and highly viscous ink, cellulose nanocrystals were combined with a silica microsphere suspension. In this method, the cellulose nanocrystals act as the carbon precursor, while the silica microspheres serve as a hard template to create macropores. Intricately designed structures were directly printed under ambient conditions, retaining their shapes with precision, as depicted in Figure 11g. After freeze-drying, carbonization, removal of the silica template, and KOH activation, the resulting aerogels exhibited multiscale pore structures of varying thicknesses (Figure 11h,i,j). The 3D-printed carbon aerogel demonstrated a capacitance of 148.6 F g-1 at a scan rate of 5 mV s-1 at -70 °C. Table 3 and Table 4 provide an overview of previous research on the gravimetric performance and areal/volumetric performance of carbon electrodes with different nanoarchitectures, respectively. The selection of carbon precursors is crucial in defining the nanostructure and functional properties of carbon materials. Biomass-derived precursors can be converted into diverse carbon nanostructures, including carbon dots, carbon nanosheets, and 3D carbon materials, depending on the synthesis conditions. Their renewable nature and inherent heteroatom-containing functional groups enable precise control over pore structures and surface chemistry through pyrolysis or hydrothermal carbonization. Synthetic polymers such as polyacrylonitrile and melamine foam are widely used for producing carbon nanofibers and carbon foams, respectively. These materials often require an additional activation process to optimize pore structures and increase surface area to improve electrochemical performance. Graphene and its derivatives, such as graphene fibers and nonwoven fabrics, are primarily synthesized from chemically derived graphene oxide, which ensures high electrical conductivity. Phenolic resins are commonly used to produce spherical carbon materials, while MOFs serve as sacrificial templates to create hierarchical carbon structures with precisely tunable pore sizes.
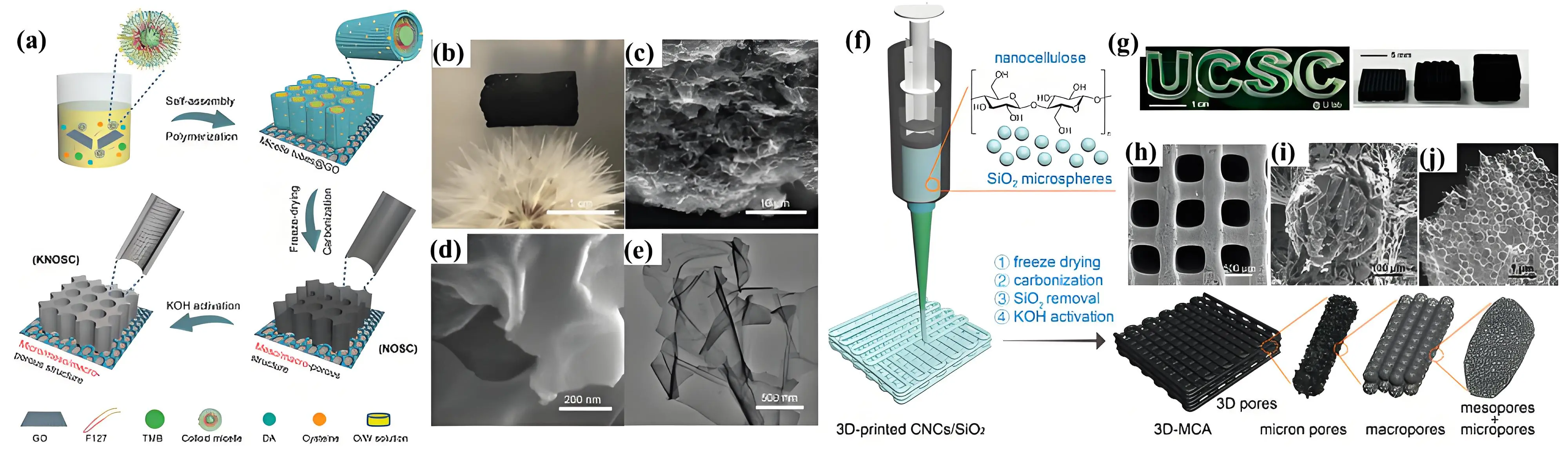
Figure 11. (a) Synthesis mechanism; (b) a digital image of N,O,S-enriched carbon aerogel sitting on a flower; (c) SEM image; (d) high-magnification SEM image; (e) TEM images of N,O,S-enriched carbon aerogel. Republished with permission from[170]; (f) synthesis mechanism, (g) digital image with a shape of "UCSC" and (h-j) top-view, cross-sectional and edge of a piece of carbon flake SEM images of 3D carbon aerogel. Republished with permission from[171]. SEM: scanning electron microscope; TEM: transmission electron microscopy; 3D: three-dimensional; GO: graphene oxide; TMB: 1,3,5-trimethylbenzene; DA: dopamine; CNCs: cellulose nanocrystals.
Samples | Surface area/m2 g-1 (pore volume/cm3 g-1) | Pore size(nm) | Heteroatoms doping (at.%) | Electrolyte | Capacitances/F g-1 (at current density/A g-1)(a) | Rate% (current density/A g-1)(a) | Energy density (Wh kg-1)(b) | Power density (kW kg-1)(b) | Device stability% (cycles) | Ref. |
GQDs | 203 (0.09) | 1.5-18 | - | 2 M KOH | 297 (1) | 54.1 (1-20) | 41.2-22.2 | 0.5-10 | 97.6 (5,000) | [99] |
N/P/O-doped carbon dots/HPC | 1,025 (0.559) | 0.7-4.0 | N (4.12) P (1.99) O (18.0) | 3 M H2SO4 3 M KOH | 510 (1) 468 (1) | 80.1 (1-30) 80.3 (1-30) | 77.3 (PbO2 as positive electrode) | 9.9 (PbO2 as positive electrode) | 91(5,000) | [100] |
GQDs-derived porous carbon | 1,323 (0.86) | 0.2-2, 5-15 | - | 6 M KOH | 315 (1) | 67 (1-50) | 9.21 | 0.247 | 100 (10,000) | [102] |
N/B doped mesoporous carbon spheres | 929 (0.96) | 3.3 | N (2.19) P (0.59) | 6 M KOH | 271 (0.5) | 80.8(0.5-10) | 7.3-4.4 | 0.21-4.24 | 96 (10,000) | [103] |
N/O-doped mesoporous carbon spheres (800 nm) | 1,321 (1.66) | 0.54, 0.86-1.27 | N (7.97) O (10.16) | 6 M KOH | 334 (1) | 61(1-20) | 9.23 | 0.4 | 93.8 (10,000) | [105] |
N/O-doped mesoporous carbon spheres (100 nm) | 1,093 (1.76) | 4.0 | N (3.26) O (4.17) | 1 M H2SO4 | 443(1) | 75.8 (1-20) | 23.4-10.9 | 0.4-14 | 91.2 (5,000) | [106] |
N-doped mesoporous carbon spheres (300 nm) | 439 (0.33) | 5.0 | N (5.33) O (5.31) | 6 M KOH | 288 (0.1) | 66 (0.1-50) | - | - | 100 (25,000) | [107] |
Ultrasmall carbon nanospheres (5 nm) | 1,689 (2.6) | 0.5-1.2, 2-6 | - | 1 M H2SO4 | 272 (0.5) | 81.6 (0.5-200) | 7.1 | 49.4 | 86.9 (10,000) | [47] |
Hollow N-doped carbon spheres (740 nm) | 1,492 (2.37) | 0.5-50 | N (3.9) | 6 M KOH 1 M H2SO4 | 254.6 (1) 284.4 (1) | 61.3 (1-10) 58.4 (1-10)) | 9.3 | 0.8 | 99.9 (10,000) | [17] |
N/P-doped hollow carbon spheres | 649 (0.51) | 0.5-3.7, 250 | N (8.03) P (0.24) | 6 M KOH | 200 (0.5) | 76 (0.5-20) | 6.4-5.1 | 0.1-2.5 | 91 (5,000) | [90] |
N-rich carbon nanofibers | 1,262 (1.04) | < 2 nm, 5-15 | N (6.41) | 6 M KOH | 203 (1) | 89.2 (1-10) | 13.95 | 0.25 | 93 (10,000) | [121] |
Hollow carbon nanofibers | 2,628 (2.32) | < 2 nm, 3-20 | - | 6 M NaOH | 330 (1) | 65.3 (1-20) | 13-10.9 | 0.6-12 | 95.3 (10,000) | [128] |
Carbon dots/ carbon nanofibers | 2,032 (1.09) | 1.1, 1.3 | N (3) | 6 M KOH | 335 (1) | 77 (1-100) | 3.73 | 34.2 | 100 (10,000) | [130] |
CNTs@hollow carbon nanofibers | 812 (1.18) | 0.5-15 | N (4.59) | 2 M KOH | 712 (1) | 67.7 (1-30) | 20.1-14.7 | 0.5-5 | 98.9 (10,000) | [131] |
Carbon nanofiber | 403 (-) | 0.7, 10-300 | N (13.5) | 6 M KOH | 338 (1) | 56 (1-20) | 11.4 | 0.34 | 95 (10,000) | [132] |
Biomass-derived carbon sheets | 1,588 (1.02) | 1-7 | O (10.68) | 1 M H2SO4 | 407 (1) | 60.4 (1-20) | 14.35 | 0.12 | 92.6 (20,000) | [147] |
Carbon sheets from biomass | 1,786 (-) | 0.5-8 | - | 6 M KOH | 447 (0.5) | 62.6 (0.5-50) | 15.5-9.7 | 0.062-6.24 | 95 (10,000) | [49] |
N/O doped carbon aerogel | 2,951 (1.84) | 0.5-0.8, 1.18-1.48 | N (1.2)O (10.1) | 6 M KOH | 401 (0.5) | 70.3 (0.5-20) | 13.3-7.86 | 0.14-2.62 | 97.3 (10,000) | [163] |
N/B doped carbon aerogel | 2,362 (1.45) | < 2, 2-4 | N (8.8) B (2.3) | 6 M KOH | 478 (0.5) | 53.9 (0.5-50) | 14.1-1.5 | 0.29-5.25 | 100 (10,0000) | [164] |
3D hierarchical porous carbon | 2,582 | 0.48-4 | N (1.27) | 1 M H2SO4 | 401 (5) | 86.3 (5-100) | 89-36.5(in TEABF4) | 99-512(in TEABF4) | - | [168] |
N/O/S doped hierarchical porous carbon foam | 2,685 (1.39) | 0.9, 2.5-35 | N (6.71) O (4.52) S (4.94) | 6 M KOH | 402.5 (1) | 76.6 (1-100) | 15.4 | 0.55 | 90 (20,000) | [170] |
(a): Specific capacitance and rate performance listed were obtained from three-electrode system; (b): E and P values listed were obtained from symmetric supercapacitors based on the carbon materials mentioned in the first column; GQDs: graphene quantum dots; HPC: hydroxypropyl cellulose; CNTs: carbon nanotubes.
Samples | Surface area/m2 g-1 | Pore size (nm) | Electrolyte | Capacitances (at current density)(a) | Rate% (current density)(a) | Energy density(b) | Power density(b) | Device stability% (cycles) | Ref. |
N/O doped GQDs | - | - | 1 M H2SO4 | 92.5 F cm-3 (1 mV s-1) | - | 7.8mWh cm-3 | 140.4 mW cm-3 | 82.6 (5,000) | [92] |
Carbon dots derived graphene | 230 | - | 0.1 M TBAPF6 | 27.5 mF dm-3 (560 mA cm-3) | - | 24.1 μWh cm-3 | 711 mW cm-3 | 94.6 (20,000) | [101] |
Graphene fibers | 356 | - | 1 M H2SO4 | 340 F cm-3 (0.244 A cm-3) | 52.4 (0.244-2.44 A cm-3) | 7.03 mWh cm-3 | 57.7 m Wcm-3 | 92 (1,000) | [138] |
Holey graphene fibers | 569 | 2.92 | 1 M H2SO4 | 2670 mF cm-2 (0.1 mA cm-2) | 71.4 (0.1-1 mA cm-2) | 335.8-136.7 μWh cm-2 | 1.5-30 mW cm-2 | 93.6 (10,000) | [139] |
CNTs/graphene hybrid fiber | 268 | 2.5-350 | 1 M H2SO4 | 62.1 F cm-3 (0.1 mA cm-3) | 67 (0.1-1 mA cm-3) | 8.63 mWh cm-3 | 2.03 W cm-3 | 97.8 (20,000) | [142] |
Carbon dots/graphene hybrid fiber | 435 | 1.2-95.3 | 1 M H2SO4 | 607 mF cm-2 (0.1 A cm-2) | 65.4 (0.1-1 mA cm-2) | 67.37-46.67 μ Wh cm-2 | 1.5-15 mW cm-2 | 95 (10,000) | [143] |
N,F co-doped Mesoporous carbon fiber | 327 | 2.5-12.7 | 1M H2SO4 | 243.9 mF cm-2 (10 μA cm-2) | 80.8 (10-100 μA cm-2) | 30.5-25.3 μWh cm-2 | 18-90 μW cm-2 | 91.3 (10,000) | [177] |
Graphene film | 480 | - | 1M H2SO4 | 225 mF cm-2 (0.1 mA cm-2) | 61 (0.1-10 mA cm-2) | 0.22-0.11 mWh cm-2 | 1-10.3 mW cm-2 | 99.4 (20,000) | [149] |
Multilayer graphene film | - | - | 3M KOH | 164 F cm-3 (0.85 A cm-3) | 46 (0.85-17 A cm-3) | 120.27 Wh L-1 | 641.4 W L-1 | 94.3 (10,000) | [151] |
Hydrothermal GFFs | 245 | 0.5-5 | 1M H2SO4 | 1,060 mF cm-2 (1 mA cm-2) | 84 (1-100 mA cm-2) | 23.5 μWh cm-2 | 26.3 mW cm-2 | 100 (50,000) | [156] |
N2H4 reduced GFFs | 65.6 | - | 1M H2SO4 | 2,812 mF cm-2 (1 mA cm-2) | 84 (1-10 mA cm-2) | 28-11 μWh cm-2 (PANI/GFF electrode) | 254-4,950 μW cm-2 | 100 (10,000) | [157] |
H2O2 activated GFFs | 221 | 1-50 | 1M H2SO4 | 1,248.5 mF cm2 (0.1 mA cm-2) | 63 (0.1-100 mA cm-2) | 35.8-102 μWh cm-2 | 58.6-1.7 mW cm-2 | 95 (60,000) | [158] |
(a): Specific capacitance and rate performance listed were obtained from three-electrode system; (b): E and P values listed were obtained from symmetric supercapacitors based on the carbon materials mentioned in the first column; GQDs: graphene quantum dots; CNTs: carbon nanotubes; CFFs: graphene fiber fabrics.
5. Practical Application of Carbon-Based Supercapacitors
Supercapacitors designed for power systems typically exhibit high power density, fast charge/discharge capability, and excellent mechanical durability. These characteristics make them ideal for powering small-scale applications such as model electric vehicles, miniature elevators, and other lightweight mechanical systems. In recent years, the practical application of supercapacitors, especially flexible ones, has gained significant attention. While maintaining the benefits of traditional supercapacitors, flexible supercapacitors offer additional advantages, including exceptional flexibility, lightweight structures, and high compressive strength. As a result, flexible supercapacitors have been widely adopted use in lightweight, flexible, and wearable electronic devices, including smart textiles with embedded electronics, medical monitoring and implantable systems[172,173]. 0D carbon materials typically require a conductive substrate and binder to establish a conductive pathway. In contrast, 1D and 2D carbon materials stand out due to their exceptional flexibility, making them ideal candidates for directly constructing flexible supercapacitors[174,175].
5.1 Wearable electronics
Micro-supercapacitors based on N-doped graphene fibers have demonstrated significant potential for powering audio-visual electronics. For instance, one such device achieved an energy density of 46.9 μWh cm-2 at a power density of 15 W cm-2 in an organic electrolyte[176]. When integrated into woven fabric and connected in parallel, two micro-supercapacitors successfully powered an audio system (Figure 12a). Similarly, fiber-shaped micro-supercapacitors mounted on flexible polyethylene glycol terephthalate, and arranged in parallel or series, generated sufficient energy to illuminate 16 light-emitting diodes (LEDs), operate a monochrome display, power a backlight, and support a multicolor display. They were also capable of efficiently running a wristwatch. The startup currents required for these applications were approximately 3 mA for LEDs, 5 mA for the wristwatch, 12 mA for the backlight, and 25 mA for the multicolor display, as summarized in Figure 12b. Additionally, Wu et al. developed a fibrous supercapacitor utilizing N,F co-doped mesoporous carbon fibers, which achieved an energy density of 30.5 μWh cm-2 at a power density of 18 μW cm-2 with a PVA/H3PO4 electrolyte[177]. Three large-sized supercapacitors (~ 60 cm in length) were connected in series and were integrated into textiles, such as an ordinary sweater, and to power LEDs, as illustrated in Figure 12c. Another example of their practical application in everyday scenarios is shown in Figure 12d.
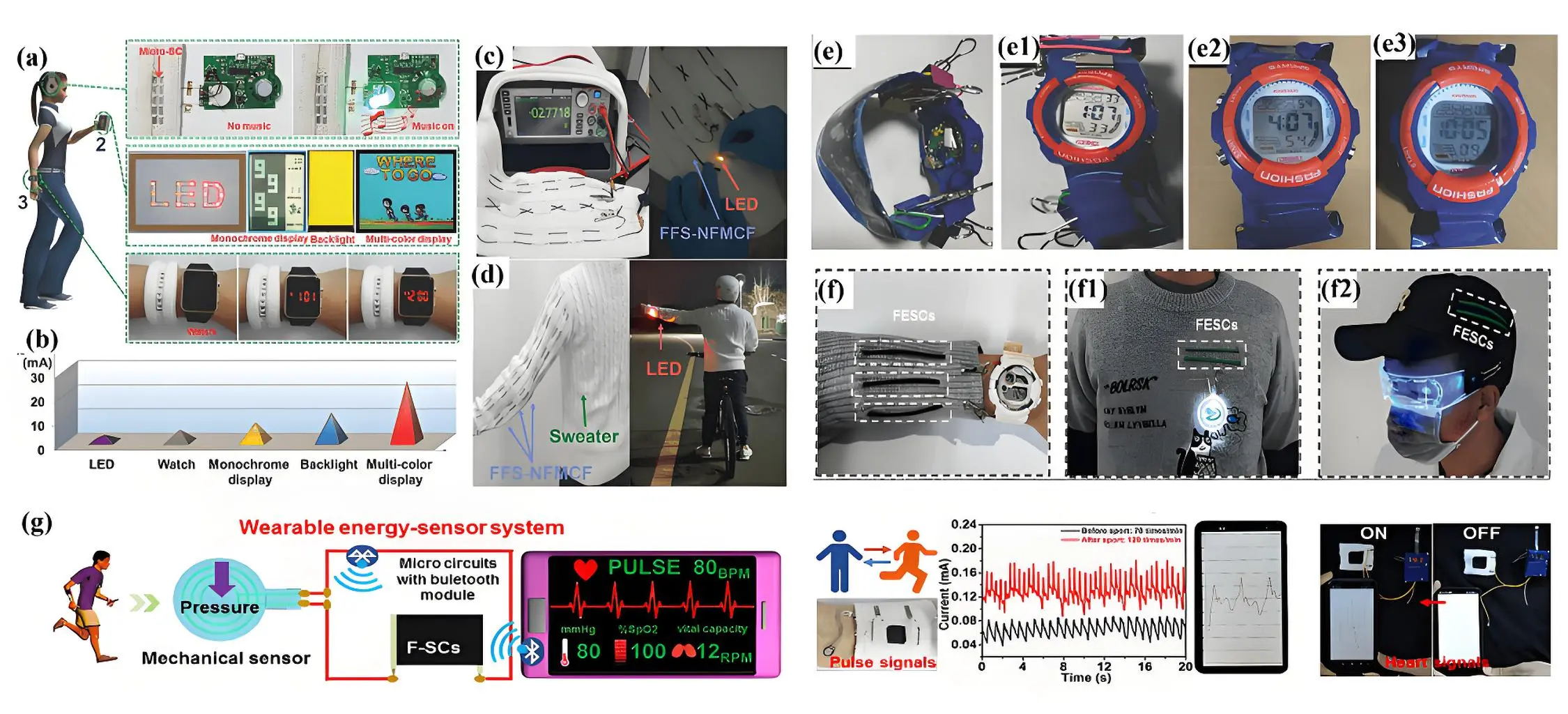
Figure 12. (a) Photographs of micro-supercapacitors integrated into woven fabrics, powering various electronics and (b) burrent output of the micro-supercapacitors for different devices. Republished with permission from[176]; (c) images of weaving of three series-connected electrodes into fabric and powering a LEDs and (d) integrated into a sweater for daily use. Republished with permission from[177]; (e) an electronic watch powered by the watchband for 10 hours. Republished with permission from[178]; (f) heterostructured polymetallic oxides and porous graphene fiber-based flexible supercapacitors powering a watch, badges, and luminous glasses. Republished with permission from[179]; (g) wearable energy sensor detecting wrist pulse and heart rate before and after exercise. Republished with permission from[184].
Carbon cloth offers greater flexibility and durability compared to fibers-based supercapacitors, making it better suited for wearable devices that require comfort and adaptability. Additionally, its woven structure provides better mechanical stability and facilitates integration into fabrics, enhancing its practicality in wearable applications. Qin et al. fabricated a carbon microfiber cloth enriched with N and O, featuring a high surface area and well-developed pore volume[178]. Symmetric fabric-based supercapacitor devices utilizing these electrodes operated within a stable voltage window of 2 V and exhibited volume energy density of ~ 9.4 mW h cm-3 at high power density of ~ 13 mW cm-3. The devices also displayed exceptional flexibility, retaining 85% of their initial capacitance after being bent to 720° for 200 cycles. Moreover, a device measuring 2 cm × 10 cm showcased its potential in practical applications by powering an electronic watch as a wristband for around nine hours (Figure 12e,e1,e2). Regarding practical applications in wearable electronics and flexible fabrics, Hu et al. systematically investigated the impressive adaptability and performance of core-sheath fibers composed of heterostructured polymetallic oxides and porous graphene fiber-based flexible supercapacitors under various conditions, such as temperature adaptability, multi-washing test, and resistance to sweat erosion[179]. The results demonstrated that the heterostructured fiber-based supercapacitor exhibits excellent temperature adaptability across a broad range (0-40 °C). Durability tests under washing conditions indicated that the supercapacitor retains 96.3% of its initial capacity after multiple washing cycles, meeting the cleaning requirements for fabric-based devices. Furthermore, the heterostructured fiber-based supercapacitors exhibit remarkable resistance to sweat erosion. Even under exposure to artificial sweat concentrations up to 100 mg cm-2, their electrochemical performance remains stable. The fibrous heterostructured graphene fiber-based flexible supercapacitors are capable of powering small wearable electronic devices, such as badges and luminous glasses (Figure 12f,f1,f2).
Wearable supercapacitors are also gaining increasing attention for integration with other device to effectively monitor human motion and enabling human-machine interface technologies[180-182]. Comfortable and intelligent flexible sensors are key contenders for the next generation of wearable electronics, as they are capable of withstanding mechanical deformation and conforming to the dynamic surfaces of human skin. The integrated system combines supercapacitor and a strain sensor. Wu et al. described the capability of a wearable system to monitor pulse signals under different conditions[183]. The system successfully recorded pulse signals from the wrist under both normal and post-exercise conditions, with real-time display capabilities on a mobile phone. Moreover, it effectively distinguished different heartbeat patterns. For instance, the signal frequency in a calm state was around 66 beats per minute, aligning closely with the typical resting heart rate of a healthy individual. Huang et al. introduced an integrated sensing system for motion monitoring and handwriting recognition[184]. The system utilized a specially engineered ionic hydrogel. This hydrogel offers excellent environmental stability, mechanical toughness, and high ion conductivity, making it highly effective as both an electrolyte for the supercapacitor and a functional component of the strain sensor. As an electrolyte for supercapacitors, the hydrogel provided a wide operating voltage range (0-2.5 V), an impressive energy density of 81.46 mWh cm-2, and exceptional deformation resistance. When employed as a strain sensor, it demonstrates reliable sensitivity across a broad strain range, along with excellent durability and repeatability. The seamless integration of these features allows the hydrogel-based strain sensor to be continuously powered by the flexible supercapacitor. This integrated system enables wireless and real-time monitoring of various physiological signals, from subtle wrist pulses to large-scale joint movements (Figure 12g). Moreover, with the incorporation of machine-learning algorithms, the system can interpret finger joint movements during handwriting and translate them into recognizable letters.
5.2 Fully self-powered device
The functionality of a fully self-powered device that integrates solar energy conversion and storage is demonstrated in a system where uses a solar cell to harvest solar energy, converting it into electrical energy, which is then stored as electrochemical energy in a flexible supercapacitor. This system can be applied to self-powered strain sensing applications and self-powered mechanical systems. The flexibility and robustness of supercapacitors allow them to seamlessly integrate into dynamic or compact designs while providing stable and reliable energy output. The B-C carbon sheet-based supercapacitors demonstrate an impressive maximum energy density of 167.05 mWh cm-3 at a power density of 0.15 W cm-3[183]. Even at a significantly higher power density of 15 W cm-3, they maintain a commendable energy density of 80 mWh cm-3. This energy storage system based on anisotropic B-carbon nanosheets, serves as a reliable power source for driving mechanical motions, as demonstrated in Figure 13a. For example, the device successfully powers a small elevator, enabling it to lift a weight up to a height of 11 cm with remarkable efficiency. Notably, the device showcases its stability by maintaining stable operation for up to 15 cycles, underscoring its potential for practical applications in small-scale mechanical systems. Qiu et al. developed hierarchical carbon polyhedron/holey graphene core-shell microfibers, achieving a maximum energy density of 335.8 μWh cm-2 at a power density of 1.5 mW cm-2[139]. Impressively, an energy density of 136.7 μWh cm-2 was maintained even at a higher power density of 30 mW cm-2. The advanced system, combining a chip-based supercapacitor, a solar cell, and an electric motor, demonstrates the feasibility of self-powered electric vehicles in a practical application. When powered solely by the solar cell, the electric car moves efficiently under sunlight but gradually comes to a stop when removed from the light source (Figure 13b). Due to the lack of an additional energy storage component, the car travels only a short distance of ~ 20 cm. In contrast, incorporating the chip-based supercapacitors into the car enables effective energy storage (Figure 13b). As a result, the car achieves a significantly extended range, traveling over 200 cm, highlighting its potential for self-powered electric mobility. Additionally, this self-sustaining system can power devices with higher resistance, such as robotic mechanisms (Figure 13c), further emphasizing its potential as a power source for intelligent robotics and other advanced technologies.
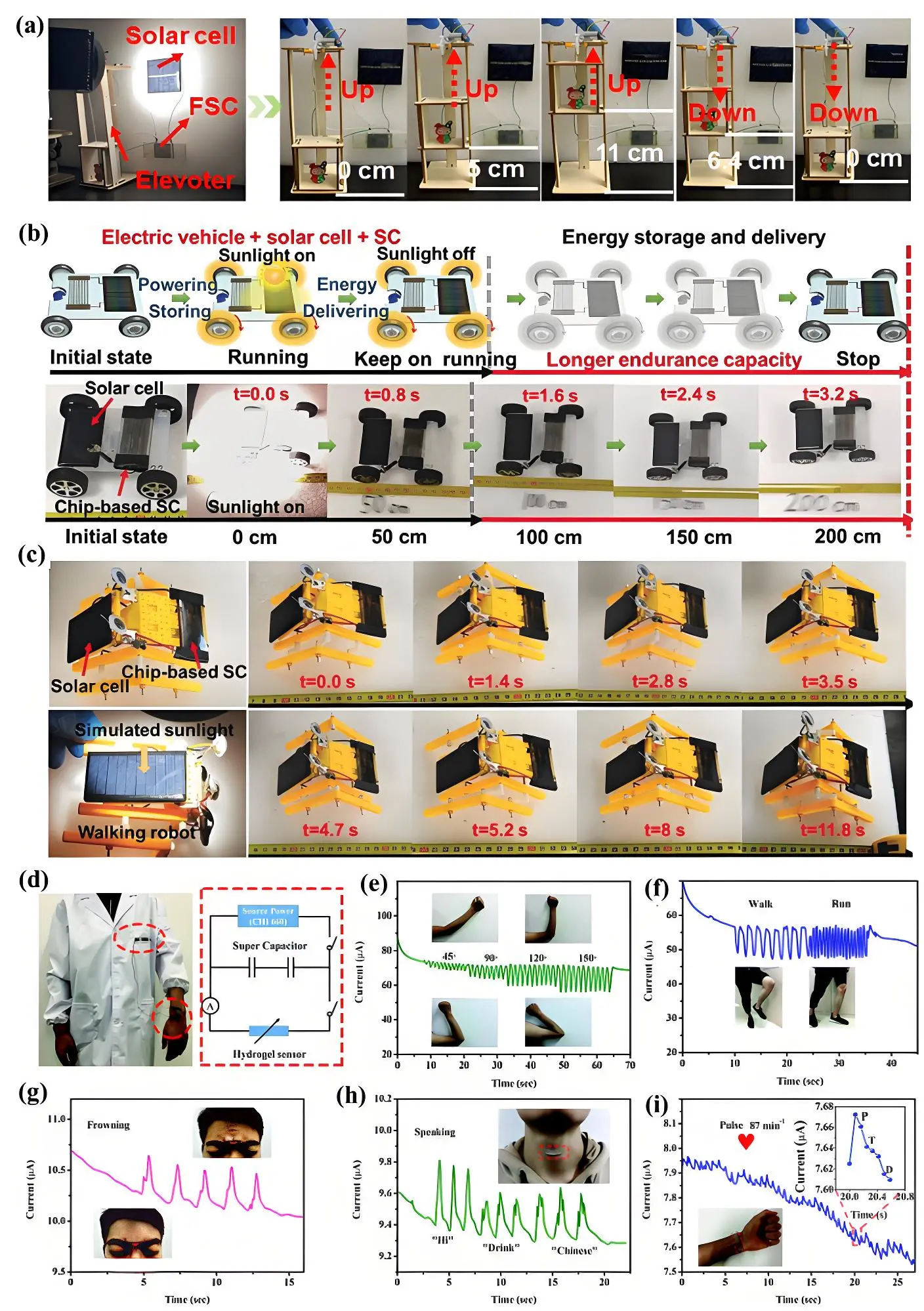
Figure 13. (a) Photographs of self-powered elevator device. Republished with permission from[184]; (b) images showcasing the movement of an electric car powered by a chip-based supercapacitor and a solar cell; (c) images of a self-powered walking robot. Republished with permission from[139]; (d) photograph of a flexible integrated system featuring a supercapacitor embedded in a coat and a sensor applied to the skin. The system's monitoring capabilities are illustrated through digital images and time-current response curves for various human motions: (e) arm movement; (f) walking/running; (g) frowning; (h) speaking; (i) wrist pulse. Republished with permission from[187].
This technology has the potential to evolve into a fully self-powered system, enabling energy-autonomous e-skin for sensors[185,186]. Huan et al. developed a flexible all-solid-state supercapacitor with an organohydrogel electrolyte to power a strain sensor[187]. The integrated fully self-powered strain sensor demonstrated highly reliability in monitoring a wide range of human movements. As shown in Figure 13d, the flexible system was mounted on the body to enable real-time motion tracking, exhibiting sensitivity to arm bending. Increasing the angle from 45° to 150° caused the sensor to stretch, leading to a noticeable rise in current output (Figure 13e). The sensor also showed consistent performance during repeated bending at fixed angles. When placed on the knee, it successfully detected both low-frequency movements like walking and high-frequency motions like running. Figure 13f,g,h,i further illustrates its ability to distinguish between motion speeds by analyzing changes in current response across different frequencies, highlighting its effectiveness in identifying similar movements at varying intensities.
5.3 Implantable devices
Supercapacitors are considered excellent candidates for biomedical applications, primarily as power sources for implantable electronic devices owing to their high power capability, long cycle life, compact size, safety, excellent biocompatibility, reliability, and environmental friendliness. Unlike rechargeable batteries, supercapacitors can charge and discharge much faster, enabling rapid energy transfer. This feature is particularly valuable for implantable devices, where a fast response time is critical, for instance, in emergency situations or when delivering treatments that require precise timing. Implantable medical devices require energy storage systems that can function safely within the human body. When implanted in vivo, these devices inevitably come into direct contact with blood, potentially triggering platelet activation, coagulation cascades, and inflammatory responses. Such adverse effects can lead to thrombosis and embolism, significantly impairing device performance and even posing life-threatening risks to patients. Therefore, beyond meeting energy storage requirements, implantable supercapacitors must exhibit exceptional anticoagulant properties to prevent thrombosis and related cardiovascular complications post-implantation. He et al. found that the hydrophilic nature of CNT surfaces played a pivotal role in promoting cell growth, as demonstrated in a comparative cell culture study conducted over one, two, and three days (Figure 14a)[188]. It was observed that cells cultured on hydrophilic CNTs exhibited a broader spindle shape, indicating enhanced biocompatibility. The oxidized CNTs-based supercapacitor demonstrated impressive durability and reliability, retaining nearly full capacitance even after 1,000 cycling (Figure 14b). Furthermore, the material exhibited excellent adaptability in biological environments like serum and blood, achieving significantly higher specific capacitances (11.4 and 13 F/g) compared to its hydrophobic counterpart (Figure 14c). Cha et al. demonstrated a significant advancement in implantable energy storage devices by using single-walled carbon nanotube (SWCNT) and oxidized SWCNT buckypaper electrodes integrated with wireless charging capabilities[189]. Oxidizing SWCNTs not only enhanced hydrophilicity and removed impurities but also improved performance in simulated physiological conditions. By assembling oxidized SWCNTs into binder-free buckypaper electrodes, the device exhibited excellent stability and biocompatibility. It achieved an energy density of 7.12 mW h cm-2 and power density of 500 mW cm-2. Notably, cytotoxicity tests confirmed the safety of electrodes over prolonged use, even after extensive charge-discharge cycles, while maintaining structural integrity due to strong material interactions. Long-term in vivo studies further validated their biostability and biocompatibility, with no signs of inflammation or tissue damage in mice over six months (Figure 14d,e,f). The seamless integration of oxidized SWCNT electrodes with a wireless charging system enabled efficient power delivery. When the supercapacitor was implanted in mice, mild-to-moderate inflammation was observed after seven days (Figure 14g). However, the minimal inflammatory response and absence of fibrotic lesions after six months were encouraging (Figure 14h). The slightly reduced inflammation in the oxidized SWCNT group highlights the potential advantage of surface modification in improving biocompatibility.
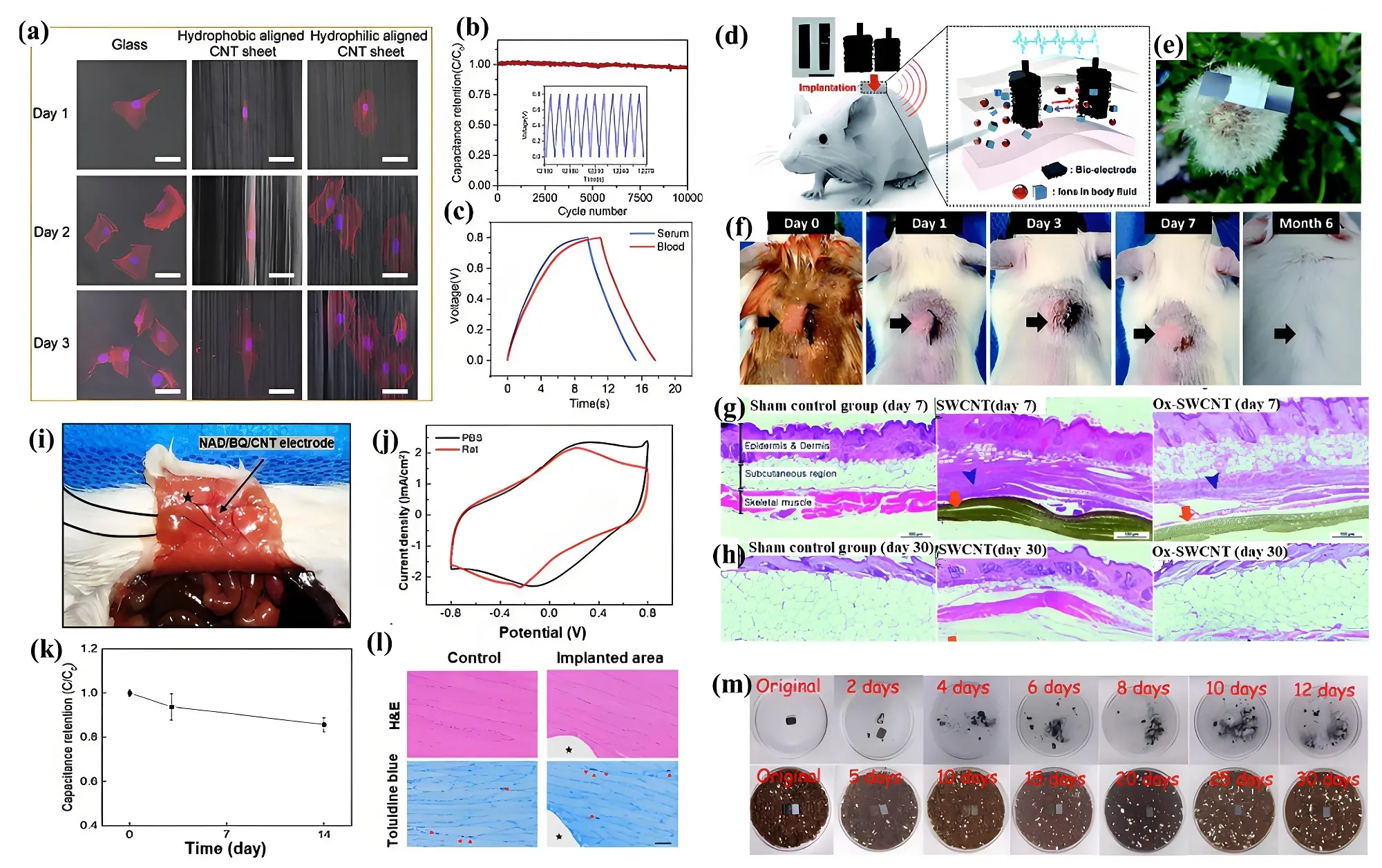
Figure 14. (a) Fluorescent images of cells cultured on different substrate; (b) CV curves of oxidized CNTs-based supercapacitor in PBS solution; (c) GCD curves of oxidized CNTs-based supercapacitor at 0.5 A cm-3 in serum and blood[188]; (d) illustration of a wireless implantable energy storage system based on Ox-SWCNT buckypaper; (e) image of the device stand on a dandelion head; (f) supercapacitors implanted in mice after (g) 7 days and (h) 6 months. Republished with permission from[189]); (i) photograph of the nicotinamide adenine dinucleotide/benzoquinone/CNT yarn supercapacitor implanted into a rat; (j) comparison of the CV curves of the supercapacitor in PBS and implanted in a rat; (k) capacitance vs time after implantation; (l) histological analysis. Republished with permission from[190]; (m) biodegradation process of the supercapacitor in gastric fluid and nutritional soil[192]. CV: cyclic voltammetry; CNTs: carbon nanotubes; PBS: phosphate-buffered saline; GCD: galvanostatic charge-discharge; SWCNT: single-walled carbon nanotube.
To further enhance the biocompatibility of devices, researchers have shifted their focus toward optimizing electrode materials. By improving their properties of these materials, they aim to develop safer and more efficient solutions for biomedical applications. Jang et al. introduced an implantable CNT yarn supercapacitor inspired by the redox mechanisms found in living cells[190]. The CNT yarn electrodes were engineered by twisting CNT sheets and electrochemically depositing nicotinamide adenine dinucleotide and benzoquinone to enable efficient redox shuttling. Under physiological conditions, such as phosphate-buffered saline and serum, these nicotinamide adenine dinucleotide/benzoquinone/CNT yarn electrodes demonstrated a remarkable maximum areal capacitance of 55.73 mF cm-2. Two flexible yarn electrodes were successfully implanted in parallel within the abdominal cavity of rats, as illustrated in Figure 14i. The supercapacitor, placed in subcutaneous tissue, demonstrated electrochemical performance comparable to its behavior in phosphate-buffered saline (PBS) solution (Figure 14j). Over a two-week monitoring period, the capacitance of the device remained stable, retaining approximately 86.9% of its initial value from the day of implantation (Figure 14k). Additionally, there was no significant difference in toluidine-positive cell counts between the control group (4.6 ± 1.4) and the implanted area, indicating minimal inflammatory response (Figure 14l). Wang et al. developed a bioelectrode material with anticoagulant functionality by incorporating heparin, a widely used clinical anticoagulant macromolecule, into the conductive polymer poly(3,4-ethylenedioxythiophene)[191]. The resulting polymer poly(3,4-ethylenedioxythiophene) incorporating heparin material was synthesized via in-situ polymerization to construct an integrated, anticoagulant supercapacitor. This innovative supercapacitor demonstrated outstanding anticoagulant performance, with a coagulation time of 63.4 seconds and a hemolysis rate below 5%. Additionally, it exhibited excellent electrochemical activity and cycling stability, making it a promising energy supply device for implantable heart rate sensors. Moreover, for advanced electronic implantable devices applications, an ideal energy storage system should inherently possess self-healing capabilities, retain stretchability after healing, and be biodegradability after use. To address this challenge, a novel self-healing, stretchable, and biodegradable supercapacitors was developed using flour as the primary component for both the electrodes and the electrolyte[192]. The biodegradability of these SCs was evaluated in simulated gastric fluid and nutrient-rich soil. Results showed complete degradation within 12 days in gastric fluid and 30 days in soil, as illustrated in Figure 14m.
6. Conclusions and Perspective
6.1 Summary
Significant advancements have been made in optimizing pore architecture, surface chemistry, electrical conductivity, and nanostructure to overcome the performance limitations of carbon-based supercapacitors. This review emphasizes the fundamental principles and recent progress in the design of carbon materials for supercapacitors, as well as their practical applications. The pore size and architecture of carbon electrodes are pivotal in determining the electrolyte contact area, thereby directly affecting specific capacitance and energy density. Micropores in the range of 0.5-2 nm and mesopore with diameter of 2-5 nm are crucial for enhancing charge storage, while large mesopores and macropores facilitate ion transport, significantly boosting rate performance. Heteroatom doping, such as with O and N, improves electrolyte wettability and increases the accessible surface area of the electrode. Elements like P, S, and B introduce pseudocapacitance, further enhancing specific capacitance. In particular, co-doping strategies, such as the combination of B and N, have shown promise in stabilizing N atoms during carbonization and amplifying pseudocapacitive contributions. However, excessive pseudocapacitive contributions can compromise cycling stability. Electrical conductivity is another key factor, critically influencing both rate performance and power density. High-conductivity materials, such as GQDs, CNTs and graphene, are frequently incorporated into composites to enhance the overall electrical properties of carbon materials. Heteroatom doping also plays a role in modulating electron mobility and concentration, further improving conductivity. Recent research has highlighted the synergistic integration of pore engineering and heteroatom doping as an effective approach for creating ultrafast supercapacitors. Additionally, large particle sizes often impede ion transport and limit the utilization of internal pore channels. To address this, tailoring the porous structure of the shell layer in carbon materials enhances electrolyte wettability, increases active site availability, and creates efficient pathways for ion and electron transport. These advancements collectively enable carbon materials to achieve high power density and excellent rate performance, even under high current densities.
Nanostructure design is a critical factor in optimizing the performance of carbon materials for supercapacitors. 0D carbon materials, such as carbon/graphene quantum dots and carbon nanospheres, offer remarkable electrical properties and structural benefits, including a high surface-to-volume ratio, excellent conductivity, and abundant active sites. The ultrasmall size of quantum dots enhances electron transfer and storage by leveraging edge quantum effects; however, the absence of a continuous conductive network restricts electron transport efficiency, and the lack of interconnected pores hinders ion diffusion, particularly in densely packed configurations, thereby limiting rate performance. 1D carbon materials, including CNTs, carbon nanofibers, and graphene fibers, enable efficient electron transport along their longitudinal axes and often possess interconnected porous structures that improve ion diffusion. Electrospun carbon nanofibers and graphene fibers are particularly notable for their tunable structures, which can be optimized through generating pores, heteroatom doping, and the incorporation of active materials to enhance their specific surface area and capacitance. Moreover, the fibrous networks formed can be directly employed as electrodes without requiring additional binders or conductive additives. Despite these advantages, 1D materials generally exhibit a lower surface area compared to 2D and 3D counterparts, which limits their energy storage capacity. Additionally, achieving uniform pore size distribution and preventing aggregation in dense assemblies remain significant challenges. 2D carbon materials, such as graphene, graphene oxide, and carbon nanosheets, effectively balance surface area and conductivity, with their layered porous structures facilitating ion diffusion by minimizing transport resistance and shortening diffusion paths, while their high conductivity ensures rapid electron transfer. However, the tendency of 2D layers to restack during processing can dramatically reduce their effective surface area and ion accessibility, while the absence of hierarchical pores in pristine 2D materials restricts electrolyte permeability and mass transport. Strategies such as pore engineering, structural modifications, and functionalization of graphene layers can alleviate these issues, and the incorporation of fillers such as carbon quantum dots or CNTs can further mitigate restacking and improve electrochemical performance. 3D carbon materials, including porous carbons, aerogels, and foams, integrate hierarchical porous structures that combine micro-, meso-, and macropores, offering a balance between ion accessibility and electrolyte transport. These materials provide both high energy density, attributed to their large surface areas, and high-power density, resulting from efficient ion and electron transport pathways. Nevertheless, their synthesis often involves complex procedures, and achieving uniform pore structures with optimal conductivity remains a challenge. Furthermore, the mechanical stability of some 3D carbon materials under prolonged cycling or high-current conditions presents an additional limitation.
6.2 Challenges
High porosity and high conductivity in carbon materials are essential for achieving high performance supercapacitors. Micropores can store more charge, while mesopores and high conductivity facilitate rapid ions movement, enhancing power density. Ions migrate through mesopores to reach micropores, and a higher mesopore content increases ion transport efficiency. High conductivity facilitates ion mobility within the material structure. Therefore, integrating both characteristics within a single material enables supercapacitors to achieve higher capacitance and improved power performance. However, these two properties are often conflict. Materials with high conductivity, such as graphene or graphite, typically possess highly ordered crystal structures, whereas materials with large surface areas often exhibit discontinuities in electron transfer. One way to overcome this challenge is by synthesizing a composite of porous carbon and highly conductive carbons (such as CNTs or graphene), which promote electron transport while maintaining porosity. Additionally, incorporating hierarchical porosity with 3D interconnected networks enhances ion diffusion pathways and electrolyte accessibility, while combining different dimensional structures (e.g., dot-line-sheet architectures) can further optimize charge storage and transport.
Although recent studies report that carbon materials can achieve high specific capacitance exceeding 500 F g-1, this performance is often achieved at the expense of electrode material loading, with typical electrode mass below 2 mg. For practical applications, commercially available supercapacitors require electrode loadings above 10 mg cm-2. Under such conditions, ion migration channels become severely obstructed, and the utilization of bulk material is significantly reduced, leading to unsatisfactory capacitance and rate performance. As the mass loading increases, most carbon materials exhibit a substantial decline in performance due to reduced conductivity and blocked ion migration pathways. Consequently, porous carbon materials must achieve a balance between excellent conductivity and stable ion migration dynamics. However, excessive porosity often disrupts the large-scale conjugated networks required for electrical conductivity, resulting in lower conductivity overall. A major challenge under high material loading is preserving efficient ion migration pathways, as thick electrodes require well-structured ion transport channels and ample electrolyte reservoirs to maintain performance. To mitigate these limitations, 2D carbon architectures significantly enhance mass transport efficiency and improve interfacial wettability, thereby expanding the accessible ion penetration domain within porous carbon electrodes. This results in simultaneous improvements in charge storage density and dynamic response rate. Building upon the enhanced ion accessibility enabled by 2D structural design, the targeted construction of highly active energy storage sites, e.g., through precise tuning of surface functional groups and gradient heteroatom doping, can effectively overcome charge transfer resistance at the electrode/electrolyte interface. This strategy enables a balance between high areal capacitance and long-term cycling stability. Furthermore, machine learning and AI-driven materials design offer powerful tools for discovering novel carbon materials with tailored properties for specific applications.
Despite significant advancements, the energy density of carbon-based supercapacitors remains low. Current research has largely focused on improving energy density through strategies such as increasing specific capacitance, widening the voltage window, or incorporating pseudocapacitive or battery-like materials. However, these approaches often come at the cost of power density or cycling stability. Without these core characteristics, supercapacitors risk losing their distinct advantages over battery-like energy storage devices. In practical applications, high-power supercapacitors are indispensable for various uses, including heavy-load operations, regenerative braking energy recovery, and load balancing in smart grids. Therefore, achieving a balance between high power density and high energy density remains a challenge. Future studies should adopt a multifaceted structural engineering approach to optimize both charge storage and ion transport properties. This includes designing hierarchical porosity to enhance ion accessibility while maintaining a high effective surface area for charge storage, introducing heteroatom doping (e.g., B, N, S, P co-doping) to create additional redox-active sites that boost electrochemical performance, and developing advanced electrode architectures (e.g., 3D printed electrodes and fiber-based electrodes) to ensure efficient ion diffusion and mechanical robustness under high mass loading.
6.3 Commercial perspective
By the end of 2021, China's supercapacitor market had grown to 17.6 billion Yuan, representing over 70% of the global market share[193,194]. The global supercapacitor market is projected to reach $5.2 billion by 2028, highlighting the growing demand for standardized guidelines to enhance electrode performance. From both commercial and scientific perspectives, carbon-based materials dominate supercapacitor electrodes due to their cost-effectiveness, high electrical conductivity, chemical stability, and environmental sustainability. Among them, activated carbon is widely used in commercial supercapacitors, benefiting from its natural abundance and well-developed porous structure for efficient charge storage, and mature production infrastructure, primarily based on biomass precursors (e.g., coconut shells, wood pulp). Its affordability ($5-20 kg-1) and exceptional cycling durability (> 100,000 cycles with < 20% capacitance fade) further reinforce its market dominance.
However, traditional carbon materials face inherent limitations in achieve high energy density without sacrificing power density or long-term stability, which restricts their application in high-energy storage systems. In response, advanced carbon materials such as graphene, CNTs, and carbon aerogels have been explored for their superior electrochemical performance. Despite their potential, the large-scale production and commercialization of advanced carbon electrodes for supercapacitors face significant economic challenges, primarily due to high production costs, energy-intensive synthesis, and scalability limitations. For instance, graphene synthesized via the Hummers method often suffers from environmental pollution and high production costs (> $200 kg-1), CNT production through CVD requires high energy input (> 800 °C) and the yield is quite low, and carbon aerogels, while demonstrating excellent performance, struggle with scalability due to the high cost of supercritical drying, which accounts for 60-70% of total manufacturing expenses. The commercialization of these high-performance carbon materials, depends on breakthroughs in scalable production and cost-effective synthesis technologies.
The accelerating adoption of supercapacitors in electric vehicles, renewable energy storage, and industrial power backup systems is driving the urgent need for cost-effective, high-performance carbon electrodes. As the market expands, investment in energy storage technologies and electrode material advancements will be crucial. To achieve economic feasibility, future research should focus on developing low-cost, scalable synthesis techniques such as biomass-derived carbons, low-energy catalytic growth, and automated 3D-printed electrode architectures.
Authors contribution
Liu L, Qin X: Conceptualization, writing-original draft.
Zhang R: Writing-review & editing.
All authors have given approval to the final version of the manuscript.
Conflicts of interest
The authors declare no conflicts of interest.
Ethical approval
Not applicable.
Consent to participate
Not applicable.
Consent for publication
Not applicable.
Availability of data and materials
Not applicable.
Funding
This work was supported by the Natural Science Foundation of Shandong Province (ZR2024ME021 and ZR2019QEM005).
Copyright
© The Author(s) 2025.
References
-
1. Wang F, Wu X, Yuan X, Liu Z, Zhang Y, Fu L, et al. Latest advances in supercapacitors: from new electrode materials to novel device design. Chem Soc Rev. 2017;46:6816-6854.
[DOI] -
2. Pramanik A, Sengupta S, Saju SK, Chattopadhyay S, Kundu M, Ajayan PM. Ternary metal sulfides as electrode materials for Na/K-Ion batteries and electrochemical supercapacitor: advances/ahallenges and prospects. Adv Energy Mater. 2024;14(36):e2401657.
[DOI] -
3. Saju SK, Chattopadhyay S, Xu J, Alhashim S, Pramanik A, Ajayan PM. Hard carbon anode for lithium-, sodium-, and potassium-ion batteries: Advancement and future perspective. Cell Rep Phys Sci. 2024;5(3):101851.
[DOI] -
4. Wang G, Zhang L, Zhang J. A review of electrode materials for electrochemical supercapacitors. Chem Soc Rev. 2012;41(2):797-828.
[DOI] -
5. Xie XC, Yu L. Recent advances in condensed-matter physics in China. Nat Sci Rev. 2017;4(2):153-154.
[DOI] -
6. Liu G, Chen X, Liu C, Jiang Q, Jiang F, An J, et al. DMSO-treated flexible PEDOT: PSS/PANi fiber electrode for high performance supercapacitors. J Mater Sci. 2021;56:14632-14643.
[DOI] -
7. Wang Y, Ding Y, Guo X, Yu G. Conductive polymers for stretchable supercapacitors. Nano Res. 2019;12:1978-1987.
[DOI] -
8. Yu X, Song S, Yi JL, Liu S, Zhang R, Wang F, et al. Facile assembly of cobalt-nickel double hydroxide nanoflakes on nitrogen-doped hollow carbon spheres for high performance asymmetric supercapacitors. J Alloy Compd. 2022;918:165551.
[DOI] -
9. Tang G, Liang J, Wu W. Transition Metal Selenides for Supercapacitors. Adv Funct Mater. 2024;34(9):2310399.
[DOI] -
10. Du J, Chen A, Liu L, Li B, Zhang Y. N-doped hollow mesoporous carbon spheres prepared by polybenzoxazines precursor for energy storage. Carbon. 2020;160:265-272.
[DOI] -
11. Li N, Qin B, Kang H, Cai N, Huang S, Xiao Q. Engineering hollow carbon spheres: directly from solid resin spheres to porous hollow carbon spheres via air-induced linker cleaving. Nanoscale. 2021;13(32):13873-13881.
[DOI] -
12. Li J, Han K, Wang D, Teng Z, Cao Y, Qi J, et al. Fabrication of high-performance structural N-doped hierarchical porous carbon for supercapacitors. Carbon. 2020;164:42-50.
[DOI] -
13. Liu M, Li X, Shao C, Han C, Liu Y, Li X, et al. Synchronous-ultrahigh conductive-reactive N-atoms doping strategy of carbon nanofibers networks for high-performance flexible energy storage. Energy Storage Mater. 2022;44:250-262.
[DOI] -
14. Ma D, Chen L, Wang G, Shen X, Yuan A, Li H, et al. Nitrogen and sulfur co-doped carbon sub-micrometer sphere-based electrodes toward high-performance hybrid supercapacitors. Appl Surf Sci. 2022;590:153121.
[DOI] -
15. Zhong M, Zhang M, Li X. Carbon nanomaterials and their composites for supercapacitors. Carbon Energy. 2022;4:950-985.
[DOI] -
16. Raja Selvaraj, Muthusamy A, Cho I, Kim HJ, Senthil K, Prabakar K. Ultrahigh surface area biomass-derived 3D hierarchical porous carbon nanosheet electrodes for high energy density supercapacitors. Carbon. 2021;174:463-474.
[DOI] -
17. Zhang J, Wu D, Zhang Q, Zhang A, Sun J, Hou L, et al. Green self-activation engineering of metal-organic framework-derived hollow nitrogen-doped carbon spheres towards supercapacitors. J Mater Chem A. 2022;10(6):2932-2944.
[DOI] -
18. Yu S, Ye Y, Yang M, Liu Y, Yang D, Li H, et al. Ammonium folate-reinforced self-assembly of gelatin into N/B/O-enriched hierarchical porous carbons with loosely layered structure for anti-freezing flexible supercapacitors. Small. 2024;20(8):2306267.
[DOI] -
19. Xu Z, Sun Z, Shan J, Jin S, Cui J, Deng Z, et al. O, N-Codoped, self-activated, holey carbon sheets for low-cost and high-loading zinc-ion supercapacitors. Adv Funct Mater. 2024;34(14):2302818.
[DOI] -
20. Edathil AA, Rezaei B, Almdal K, Keller SS. In situ mineralization of biomass-derived hydrogels boosts capacitive electrochemical energy storage in free-standing 3D carbon aerogels. Energy Environ Mater. 2024;7(2):e12591.
[DOI] -
21. Guan T, Li Z, Qiu D, Wu G, Wu J, Zhu L, et al. Recent progress of graphene fiber/fabric supercapacitors: from building block architecture, fiber assembly, and fabric construction to wearable applications. Adv Fiber Mater. 2023;5:896-927.
[DOI] -
22. Jiang M, Sheng L, Wang C, Jiang L, Fan Z. Graphene film for supercapacitors: preparation, foundational unit structure, and surface regulation. Acta Phys Chim Sin. 2022;38(2):2012085.
[DOI] -
23. Shen Y, Yang J. Progress in the synthesis of carbon aerogels for advanced energy storage applications. Green Chem. 2024;26:8969-9004.
[DOI] -
24. Kumar S, Saeed G, Zhu L, Hui KN, Kim NH, Lee JH. 0D to 3D carbon-based networks combined with pseudocapacitive electrode materials for high energy density supercapacitors: a review. Chem Eng J. 2021;403:126352.
[DOI] -
25. Zhang H, Yang D, Lau A, Ma T, Lin H, Jia B. Hybridized graphene for supercapacitors: beyond the limitation of pure graphene. Small. 2021;17(12):2007311.
[DOI] -
26. Mathis TS, Kurra N, Wang X, Pinto D, Simon P, Gogotsi Y. Energy storage data reporting in perspective-guidelines for interpreting the performance of electrochemical energy storage systems. Adv Energy Mater. 2019;9(39):1902007.
[DOI] -
27. Ji H, Zhao X, Qiao Z, Jung J, Zhu Y, Lu Y, et al. Capacitance of carbon-based electrical double-layer capacitors. Nat Commun. 2014;5:3317.
[DOI] -
28. Jiang Y, Li J, Jiang Z, Shi M, Sheng R, Liu Z, et al. Large-surface-area activated carbon with high density by electrostatic densification for supercapacitor electrodes. Carbon. 2021;175:281-288.
[DOI] -
29. Chen Z, Zhao S, Zhao H, Zou Y, Yu C, Zhong W. Nitrogen-doped interpenetrating porous carbon/graphene networks for supercapacitor applications. Chem Eng J. 2021;409:127891.
[DOI] -
30. Zhang W, Yang W, Zhou H, Zhang Z, Zhao M, Liu Q, et al. Self-discharge of supercapacitors based on carbon nanotubes with different diameters. Electrochim Acta. 2020;357:136855.
[DOI] -
31. Kang J, Zhang S, Zhang Z. Three-dimensional binder-free nanoarchitectures for advanced pseudocapacitors. Adv Mater. 2017;29(48):1700515.
[DOI] -
32. Wang T, Yu F, Wang X, Xi S, Chen K, Wang S. Enhancing cycling stability of transition metal-based layered double hydroxides through a self-sacrificial strategy for hybrid supercapacitors. Electrochim Acta. 2020;334:135586.
[DOI] -
33. Liu R, Zhou A, Zhang X, Mu J, Che H, Wang Y, et al. Fundamentals, advances and challenges of transition metal compounds-based supercapacitors. Chem Eng J. 2021;412:128611.
[DOI] -
34. Wang T, Chen HC, Yu F, Zhao XS, Wang H. Boosting the cycling stability of transition metal compounds-based supercapacitors. Energy Storage Mater. 2019;16:545-573.
[DOI] -
35. Zuo W, Li R, Zhou C, Li Y, Xia J, Liu J. Battery-supercapacitor hybrid devices: recent progress and future prospects. Adv Sci. 2017;4(7):1600539.
[DOI] -
36. Chatterjee DP, Nandi AK. A review on the recent advances in hybrid supercapacitors. J Mater Chem A. 2021;9(29):15880-15918.
[DOI] -
37. Zou K, Deng W, Cai P, Deng X, Wang B, Liu C, et al. Prelithiation/presodiation techniques for advanced electrochemical energy storage systems: concepts, applications, and perspectives. Adv Funct Mater. 2021;31(5):2005581.
[DOI] -
38. Deng X, Zou K, Cai P, Wang B, Hou H, Zou J, et al. Advanced battery-type anode materials for high-performance sodium-ion capacitors. Small Methods. 2020;4(10):2000401.
[DOI] -
39. Chen M, Wang L, Sheng X, Wang T, Zhou J, Li S, et al. An ultrastable nonaqueous potassium-ion hybrid capacitor. Adv Funct Mater. 2020;30(40):2004247.
[DOI] -
40. Cai P, Wang K, Wang T, Li H, Zhou M, Wang W, et al. Comprehensive insights into potassium-Ion capacitors: mechanisms, materials, devices and future perspectives. Adv Energy Mater. 2024;14(29):2401183.
[DOI] -
41. Zhang L, Yang X, Zhang F, Long G, Zhang T, Leng K, et al. Controlling the effective surface area and pore size distribution of sp2 carbon materials and their impact on the capacitance performance of these materials. J Am Chem Soc. 2013;135(15):5921-5929.
[DOI] -
42. Chmiola J, Yushin G, Gogotsi Y, Portet C, Simon P, Taberna PL. Anomalous increase in carbon capacitance at pore sizes less than 1 nanometer. Science. 2006;313(5794):1760-1763.
[DOI] -
43. Liu YM, Merlet C, Smit B. Carbons with regular pore geometry yield fundamental insights into supercapacitor charge storage. ACS Cent Sci. 2019;5(11):1813-1823.
[DOI] -
44. Wei X, Jiang X, Wei J, Gao S. Functional groups and pore size distribution do matter to hierarchically porous carbons as high-rate-performance supercapacitors. Chem Mater. 2016;28(2):445-458.
[DOI] -
45. Wei X, Li Y, Gao S. Biomass-derived interconnected carbon nanoring electrochemical capacitors with high performance in both strongly acidic and alkaline electrolytes. J Mater Chem A. 2017;5(1):181-188.
[DOI] -
46. Huang J, Qiao R, Sumpter BG, Meunier V. Effect of diffuse layer and pore shapes in mesoporous carbon supercapacitors. J Mater Res. 2010;25(8):1469-1475.
[DOI] -
47. Liu X, Vadiyar MM, Oh JK, Ye Z. Designing ultrasmall carbon nanospheres with tailored sizes and textural properties for high-rate high-energy supercapacitors. ACS Appl Mater Interfaces. 2021;13(28):32916-32929.
[DOI] -
48. Wei X, Zou H, Gao S. Chemical crosslinking engineered nitrogen-doped carbon aerogels from polyaniline-boric acid-polyvinyl alcohol gels for high-performance electrochemical capacitors. Carbon. 2017;123:471-480.
[DOI] -
49. Zhang Y, Wu C, Dai S, Liu L, Zhang H, Shen W, et al. Rationally tuning ratio of micro- to meso-pores of biomass-derived ultrathin carbon sheets toward supercapacitors with high energy and high power density. J Colloid Interface Sci. 2022;606:817-825.
[DOI] -
50. Bleda-Martínez MJ, Maciá-Agulló JA, Lozano-Castelló D, Morallón E, Cazorla-Amorós D, Linares-Solano A. Role of surface chemistry on electric double layer capacitance of carbon materials. Carbon. 2005;43(13):2677-2684.
[DOI] -
51. Hulicova-Jurcakova D, Seredych M, Lu GQ, Bandosz TJ. Combined effect of nitrogen- and oxygen-containing functional groups of microporous activated carbon on its electrochemical performance in supercapacitors. Adv Funct Mater. 2009;19(3):438-447.
[DOI] -
52. Wang DW, Li F, Yin LC, Lu X, Chen ZG, Gentle IR, et al. Nitrogen-doped carbon monolith for alkaline supercapacitors and understanding nitrogen-induced redox transitions. Chem Eur J. 2012;18(17):5345-5351.
[DOI] -
53. Béguin F, Szostak K, Lota G, Frackowiak E. A self-supporting electrode for supercapacitors prepared by one-step pyrolysis of carbon nanotube/polyacrylonitrile blends. Adv Mater. 2005;17(19):2380-2384.
[DOI] -
54. Zhou Y, Jia Z, Shi L, Wu Z, Jie B, Zhao S, et al. Pressure difference-induced synthesis of P-doped carbon nanobowls for high-performance supercapacitors. Chem Eng J. 2020;385:123858.
[DOI] -
55. Sun P, Huang J, Xu F, Xu J, Lin T, Zhao W, et al. Boron-induced nitrogen fixation in 3D carbon materials for supercapacitors. ACS Appl Mater Interfaces. 2020;12(25):28075-28082.
[DOI] -
56. Chu Q, Chen Z, Cui C, Zhang Y, Li X, Liu G, et al. Pyrrolic-N/C=O cooperative assisted in hollow porous carbon with ultra-high electrochemical performance for Zn-ion hybrid supercapacitors. Appl Surf Sci. 2024;654:159461.
[DOI] -
57. Wang L, Men J, Feng J, Jiang Y, Li L, Hu Y, et al. Extrusion 3D printing of carbon nanotube-assembled carbon aerogel nanocomposites with high electrical conductivity. Nano Mater Sci. 2024;6(3):312-319.
[DOI] -
58. Li Q, Wang T, Shu T, Pan X, Tao Y. Controllable construction of a 3D-honeycomb-like porous carbon network as a high-performance cathode for promoting Zn-ion storage capability. Nanoscale. 2024;16(40):19086-19099.
[DOI] -
59. Li P, Liu Y, Shi S, Xu Z, Ma W, Wang Z, et al. Highly crystalline graphene fibers with superior strength and conductivities by plasticization spinning. Adv Funct Mater. 2020;30(52):2006584.
[DOI] -
60. Tang P, Deng Z, Zhang Y, Liu LX, Wang Z, Yu ZZ, et al. Tough, strong, and conductive graphene fibers by optimizing surface chemistry of graphene oxide precursor. Adv Funct Mater. 2022;32(28):2112156.
[DOI] -
61. Feng LJ, Yan JY, Bian SW. Micro/mesoporous carbon nanospheres with homogeneously N, S, P-tridoped skeletons as electrodes for high-performance supercapacitors. ACS Appl Electron Mater. 2023;5(11):6086-6093.
[DOI] -
62. Hajibaba S, Gholipour S, Pourjafarabadi M, Bakhshayesh AM, Byranvand MM, Saliba M, et al. Electrochemical sulfur-doping as an efficient method for capacitance enhancement in carbon-based supercapacitors. J Energy Storage. 2024;79:110044.
[DOI] -
63. Ke CC, Zhang N, Liu F, Yu Q, Wang FY, Liu L, et al. Deflated balloon-like nitrogen-rich sulfur-containing hierarchical porous carbons for high-rate supercapacitors. Appl Surf Sci. 2019;484:716-725.
[DOI] -
64. Lian Y, Yu G, Lu L, Guo H, Wang J, Dai Y, et al. Controllable thickness carbon sheet under anion and cation co-doping for supercapacitors and capacitive deionization. Carbon. 2024;225:119097.
[DOI] -
65. Chang Y, Shi H, Yan X, Zhang G, Chen L. A ternary B, N, P-Doped carbon material with suppressed water splitting activity for high-energy aqueous supercapacitors. Carbon. 2020;170:127-136.
[DOI] -
66. Liu Y, Ma Z, Chen X, Ying Y, Shi W. In-situ generated NiCo2O4/CoP polyhedron with rich oxygen vacancies interpenetrating by P-doped carbon nanotubes for high performance supercapacitors. J Colloid Interface Sci. 2022;608(Part 3):2246-2256.
[DOI] -
67. Liu WW, Feng YQ, Yan XB, Chen JT, Xue QJ. Superior micro-supercapacitors based on graphene quantum dots. Adv Funct Mater. 2013;23(33):4111-4122.
[DOI] -
68. Jin Y, Wang Y, Ren PG, Zhang B, Zhao Z, Hou X, et al. Recent advances of carbon dots based emerging materials for supercapacitors applications. J Energy Storage. 2024;85:111118.
[DOI] -
69. Liang J, Jiang C, Wu W. Toward fiber-, paper-, and foam-based flexible solid-state supercapacitors: electrode materials and device designs. Nanoscale. 2019;11(15):7041-7061.
[DOI] -
70. Chen D, Jiang K, Huang T, Shen G. Recent advances in fiber supercapacitors: materials, device configurations, and applications. Adv Mater. 2020;32(5):1901806.
[DOI] -
71. Yao L, Wu Q, Zhang P, Zhang J, Wang D, Li Y, et al. Scalable 2D hierarchical porous carbon nanosheets for flexible supercapacitors with ultrahigh energy density. Adv Mater. 2018;30(11):1706054.
[DOI] -
72. Liu Z, Xiong H, Luo Y, Zhang L, Hu K, Zhang L, et al. Interface-induced self-assembly strategy toward 2D ordered mesoporous carbon/MXene heterostructures for high-performance supercapacitors. ChemSusChem. 2021;14(20):4422-4430.
[DOI] -
73. Shen Z, Du J, Mo Y, Chen A. Nanocomposites of reduced graphene oxide modified with mesoporous carbon layers anchored by hollow carbon spheres for energy storage. Carbon. 2021;173:22-30.
[DOI] -
74. Wen F, Yan Y, Sun S, Li X, He X, Meng Q, et al. Synergistic effect of nitrogen and oxygen dopants in 3D hierarchical porous carbon cathodes for ultra-fast zinc ion hybrid supercapacitors. J Colloid Interface Sci. 2023;640:1029-1039.
[DOI] -
75. Liu W, Mei J, Liu G, Kou Q, Yi T, Xiao S. Nitrogen-doped hierarchical porous carbon from wheat straw for supercapacitors. ACS Sustainable Chem Eng. 2018;6(9):11595-11605.
[DOI] -
76. Ma C, Song Y, Shi J, Zhang D, Zhai X, Zhong M, et al. Preparation and one-step activation of microporous carbon nanofibers for use as supercapacitor electrodes. Carbon. 2013;51:290-300.
[DOI] -
77. Feng P, Wang H, Huang P, Zhong L, Gan S, Wang W, et al. Nitrogen-doped lignin-derived porous carbons for supercapacitors: Effect of nanoporous structure. Chem Eng J. 2023;471:144817.
[DOI] -
78. Lv S, Ma L, Shen X, Tong H. Dual pore-former method to prepare nitrogen-doped hierarchical porous carbons for supercapacitors. J Alloys Compounds. 2022;895:162587.
[DOI] -
79. Mostazo-López MJ, Ruiz-Rosas R, Castro-Muñiz A, Nishihara H, Kyotani T, Morallón E, et al. Ultraporous nitrogen-doped zeolite-templated carbon for high power density aqueous-based supercapacitors. Carbon. 2018;129:510-519.
[DOI] -
80. Liu B, Zhang Q, Wang Z, Li L, Jin Z, Wang C, et al. Nitrogen and sulfur-codoped porous carbon nanospheres with hierarchical micromesoporous structures and an ultralarge pore volume for high-performance supercapacitors. ACS Appl Mater Interfaces. 2020;12(7):8225-8232.
[DOI] -
81. Bu L, Kuai X, Zhu W, Huang X, Tian K, Lu H, et al. Nitrogen-doped double-shell hollow carbon spheres for fast and stable sodium ion storage. Electrochim Acta. 2020;356:136804.
[DOI] -
82. Du J, Zhang Y, Wu H, Hou S, Chen A. N-doped hollow mesoporous carbon spheres by improved dissolution-capture for supercapacitors. Carbon. 2020;156:523-528.
[DOI] -
83. Shang Y, Hu X, Li X, Cai S, Li G, Zhao G, et al. A facile synthesis of nitrogen-doped hierarchical porous carbon with hollow sphere structure for high-performance supercapacitors. J Mater Sci. 2019;54(19):12747-12757.
[DOI] -
84. Liu F, Yuan RL, Zhang N, Ke CC, Ma SX, Zhang RL, et al. Solvent-induced synthesis of nitrogen-doped hollow carbon spheres with tunable surface morphology for supercapacitors. Appl Surf Sci. 2018;437:271-280.
[DOI] -
85. Yue Y, Huang YL, Bian SW. Nitrogen-doped hierarchical porous carbon films derived from metal-organic framework/cotton composite fabrics as freestanding electrodes for flexible supercapacitors. ACS Appl Electron Mater. 2021;3(5):2178-2186.
[DOI] -
86. Tang Y, Wang X, Chen J, Wang X, Wang Y, Mao Z. Templated transformation of g-C3N4 nanosheets into nitrogen-doped hollow carbon sphere with tunable nitrogen-doping properties for application in Li-ions batteries. Carbon. 2020;168:458-467.
[DOI] -
87. Wang DG, Wang H, Lin Y, Yu G, Song M, Zhong W, et al. Synthesis and morphology evolution of ultrahigh content nitrogen-doped, micropore-dominated carbon materials as high-performance supercapacitors. Chem Sus Chem. 2018;11(22):3932-3940.
[DOI] -
88. Wang C, Zhang SH, Zhang L, Xi R, Jiang DP, Chen ZY, et al. Natural bamboo leaves derived sulfur-doped mesoporous heteroatom-enriched carbon for high-performance supercapacitors and gas sensors. J Power Sources. 2019;443:227183.
[DOI] -
89. Wu M, Zhao X, Gao J, Guo J, Xiao J, Chen R. Multifunctional boron-doped carbon fiber electrodes synthesized by electrospinning for supercapacitors, dye-sensitized solar cells, and photocapacitors. Surf Interfaces. 2022;31:101983.
[DOI] -
90. Zhang N, Liu F, Xu SD, Wang FY, Yu Q, Liu L. Nitrogen-phosphorus co-doped hollow carbon microspheres with hierarchical micro-meso-macroporous shells as efficient electrodes for supercapacitors. J Mater Chem A. 2017;5(43):22631-22640.
[DOI] -
91. Lv B, Li P, Liu Y, Lin S, Gao B, Lin B. Nitrogen and phosphorus co-doped carbon hollow spheres derived from polypyrrole for high-performance supercapacitor electrodes. Appl Surf Sci. 2018;437:169-175.
[DOI] -
92. Li Z, Cao L, Qin P, Liu X, Chen Z, Wang L, et al. Nitrogen and oxygen co-doped graphene quantum dots with high capacitance performance for micro-supercapacitors. Carbon. 2018;139:67-75.
[DOI] -
93. Ni D, Sun W, Wang Z, Bai Y, Lei H, Lai X, et al. Heteroatom-doped mesoporous hollow carbon spheres for fast sodium storage with an ultralong cycle life. Adv Energy Mater. 2019;9(19):1900036.
[DOI] -
94. Liu H, Fan W, Lv H, Zhang W, Shi J, Huang M, et al. N,P-Doped carbon-based freestanding electrodes enabled by cellulose nanofibers for superior asymmetric supercapacitors. ACS Appl Energy Mater. 2021;4(3):2327-2338.
[DOI] -
95. Zhu S, Dong X, Huang H, Qi M. Rich nitrogen-doped carbon on carbon nanotubes for high-performance sodium-ion supercapacitors. J Power Sources. 2020;459:228104.
[DOI] -
96. Li X, Rui M, Song J, Shen Z, Zeng H. Carbon and graphene quantum dots for optoelectronic and energy devices: A review. Adv Funct Mater. 2015;25(31):4929-4947.
[DOI] -
97. Jin S, Allam O, Lee K, Lim J, Lee MJ, Loh SH, et al. Carbon quantum dot-modified reduced graphene oxide framework for improved alkali metal ion storage performance. Small. 2022;18(35):2202898.
[DOI] -
98. Tang T, Yuan R, Guo N, Zhu J, Gan X, et al. Improving the surface area of metal-organic framework-derived porous carbon through constructing inner support by compatible graphene quantum dots. J Colloid Interface Sci. 2022;623:77-85.
[DOI] -
99. Zhang S, Sui L, Dong H, He W, Dong L, Yu L. High-performance supercapacitor of graphene quantum dots with uniform sizes. ACS Appl Mater Interfaces. 2018;10(15):12983-12991.
[DOI] -
100. Wei JS, Ding C, Zhang P, Ding H, Niu XQ, Ma YY, et al. Robust negative electrode materials derived from carbon dots and porous hydrogels for high-performance hybrid supercapacitors. Adv Mater. 2018;31(5):1806197.
[DOI] -
101. Strauss V, Marsh K, Kowal MD, El-Kady M, Kaner RB. A simple route to porous graphene from carbon nanodots for supercapacitor applications. Adv Mater. 2018;30(8):1704449.
[DOI] -
102. Tian W, Zhu J, Dong Y, Zhao J, Li J, Guo N, et al. Micelle-induced assembly of graphene quantum dots into conductive porous carbon for high rate supercapacitor electrodes at high mass loadings. Carbon. 2020;161:89-96.
[DOI] -
103. Du J, Zhang Y, Lv H, Chen A. N/B-co-doped ordered mesoporous carbon spheres by ionothermal strategy for enhancing supercapacitor performance. J Colloid Interf Sci. 2021;587:780-788.
[DOI] -
104. Yang X, Li Y, Zhang P, Sun L, Ren X, Mi H. Hierarchical hollow carbon spheres: novel synthesis strategy, pore structure engineering and application for micro-supercapacitor. Carbon. 2020;157:70-79.
[DOI] -
105. Xue D, Zhu D, Xiong W, Cao T, Wang Z, Lv Y, et al. Template-free, self-doped approach to porous carbon spheres with high N/O contents for high-performance supercapacitors. ACS Sustain Chem Eng. 2019;7(7):7024-7034.
[DOI] -
106. Qiu Y, Hou M, Gao J, Zhai H, Liu H, Jin M, et al. One-step synthesis of monodispersed mesoporous carbon nanospheres for high-performance flexible quasi-solid-state micro-supercapacitors. Small. 2019;15(45):903836.
[DOI] -
107. Wang JG, Liu H, Sun H, Hua W, Wang H, Liu X, et al. One-pot synthesis of nitrogen-doped ordered mesoporous carbon spheres for high-rate and long-cycle life supercapacitors. Carbon. 2018;127:85-92.
[DOI] -
108. Ren FX, Wang HW, Wang Q, Qiu R, Tang S, Wang R, et al. In situ hard-template synthesis of hollow bowl-like carbon: a potential versatile platform for sodium and zinc ion capacitors. Adv Energy Mater. 2020;10(47):2002741.
[DOI] -
109. Zhou Y, Jia Z, Zhao S, Chen P, Guo Y, Wang T, et al. Construction of triple-shelled hollow nanostructure by confining amorphous Ni-Co-S/crystalline MnS on/in hollow carbon nanospheres for all-solid-state hybrid supercapacitors. Chem Eng J. 2021;416:129500.
[DOI] -
110. Chen L, Yin H, Wu Z, Liu H, Du W, Jing Y, et al. Non-etching and controllable fabrication of hollow carbon spheres for high-performance supercapacitor. J Energy Storage. 2023;73:109111.
[DOI] -
111. Zhang M, Jiang S, Zou J, Qu X, Zhang Z, Wang R, et al. N-doped yolk-shell carbon nanospheres with "carbon bridges" for supercapacitors. ACS Appl Nano Mater. 2023;6(10):8279-8289.
[DOI] -
112. Niu C, Sichel EK, Hoch R, Moy D, Tennent H. High-power electrochemical capacitors based on carbon nanotube electrodes. Appl Phys Lett. 1997;70(11):1480-1482.
[DOI] -
113. John AR, Arumugam P. Open ended nitrogen-doped carbon nanotubes for the electrochemical storage of energy in a supercapacitor electrode. J Power Sources. 2015;277:387-392.
[DOI] -
114. Frackowiak E, Delpeux S, Jurewicz K, Szostak K, Cazorla-Amoros D, Beguin F. Enhanced capacitance of carbon nanotubes through chemical activation. Chem Phys Lett. 2002;361(1-2):35-41.
[DOI] -
115. Zhang Q, Huang JQ, Qian WZ, Zhang YY, Wei F. The road for nanomaterials industry: a review of carbon nanotube production, post-treatment, and bulk applications for composites and energy storage. Small. 2013;9(8):1237-1265.
[DOI] -
116. Sun L, Wang X, Wang Y, Zhang Q. Roles of carbon nanotubes in novel energy storage devices. Carbon. 2017;122:462-474.
[DOI] -
117. Liu Y, Wang Y, Nie Y, Wang C, Zhou L, et al. Preparation of MWCNTs-graphene-cellulose fiber with ionic liquids. ACS Sustainable Chem Eng. 2019;7(24):20013-20021.
[DOI] -
118. Xia S, Zhang Y, Zhao Y, Wang X, Yan J. Hierarchical porous carbon nanofibers with tunable geometries and porous structures fabricated by a scalable electrospinning technique. ACS Appl Mater Interfaces. 2021;13(37):44768-44776.
[DOI] -
119. Chen S, Qiu L, Cheng HM. Carbon-based fibers for advanced electrochemical energy storage devices. Chem Rev. 2020;120(5):2811-2878.
[DOI] -
120. Kim C, Yang KS. Electrochemical properties of carbon nanofiber web as an electrode for supercapacitor prepared by electrospinning. Appl Phys Lett. 2003;83(6):1216-1218.
[DOI] -
121. Amiri A, Conlee B, Tallerine I, Kennedy WJ, Naraghi M. A novel path towards synthesis of nitrogen-rich porous carbon nanofibers for high performance supercapacitors. Chem Eng J. 2020;399:125788.
[DOI] -
122. Zhu J, Zhang Q, Guo L, Zhao Y, Zhang R, Liu L, et al. Highly flexible, freestanding supercapacitor electrodes based on hollow hierarchical porous carbon nanofibers bridged by carbon nanotubes. Chem Eng J. 2022;434:134662.
[DOI] -
123. Liu J, Xiong Z, Wang S, Cai W, Yang J, Zhang H. Structure and electrochemistry comparison of electrospun porous carbon nanofibers for capacitive deionization. Electrochim Acta. 2016;210:171-180.
[DOI] -
124. Ji L, Lin Z, Medford AJ, Zhang X. Porous carbon nanofibers from electrospun polyacrylonitrile/SiO2 composites as an energy storage material. Carbon. 2009;47(14):3346-3354.
[DOI] -
125. Zhou Z, Liu G. Controlling the pore size of mesoporous carbon thin films through thermal and solvent annealing. Small. 2017;13(15):1603107.
[DOI] -
126. Zhou Z, Liu T, Khan AU, Liu G. Block copolymer-based porous carbon fibers. Sci Adv. 2019;5(2):685.
[DOI] -
127. Peng L, Zhang X, Sun Y, Li C. Electrospun ZIF-derived cavity porous carbon nanofibers as a freestanding cathode for lithium-oxygen batteries with ultralow overpotential. Nanoscale. 2021;13(39):16477-16486.
[DOI] -
128. Liu Y, Liu Q, Wang L, Yang X, Yang W, Zheng J, et al. Advanced supercapacitors based on porous hollow carbon nanofiber electrodes with high specific capacitance and large energy density. ACS Appl Mater Interfaces. 2020;12(4):4777-4786.
[DOI] -
129. Zhu J, Zhang S, Wang L, Jia D, Xu M, Zhao Z, et al. Engineering cross-linking by coal-based graphene quantum dots toward tough, flexible, and hydrophobic electrospun carbon nanofiber fabrics. Carbon. 2018;129:54-62.
[DOI] -
130. Zhao J, Zhu J, Li Y, Wang L, Dong Y, Jiang Z, et al. Graphene quantum dot reinforced electrospun carbon nanofiber fabrics with high surface area for ultrahigh rate supercapacitors. ACS Appl Mater Interfaces. 2020;12(10):11669-11678.
[DOI] -
131. Kim T, Subedi S, Dahal B, Chhetri K, Mukhiya T, Muthurasu A, et al. Homogeneous elongation of N-doped CNTs over nano-fibrillated hollow-carbon-nanofiber: mass and charge balance in asymmetric supercapacitors is no longer problematic. Adv Sci. 2022;9(20):2200650.
[DOI] -
132. Mun WJ, Kim B, Moon SJ, Kim JH. Hydrogen-bonded organic framework-derived, flower-on-fiber-like, carbon nanofiber electrodes for supercapacitors. J Mater Chem A. 2024;12(11):6712-6723.
[DOI] -
133. Xu Z, Gao C. Graphene fiber: A new trend in carbon fibers. Mater Today. 2015;18(9):480-492.
[DOI] -
134. Yang M, Wang Z, LI P, Liu Y, Lin J, Wang B, et al. Stress relaxation behaviors of graphene fibers. Carbon. 2021;182:384-392.
[DOI] -
135. Gopalsamy K, Yang Q, Cai S, Huang T, Gao Z, Gao C. Wet-spun poly(ionic liquid)-graphene hybrid fibers for high performance all-solid-state flexible supercapacitors. J Energy Chem. 2019;34:104-110.
[DOI] -
136. Jiang Y, Wang Y, Xu Z, Gao C. Conformation engineering of two-dimensional macromolecules: a case study with graphene oxide. Acc Mater Res. 2020;1(3):175-187.
[DOI] -
137. Qu G, Cheng J, Li X, Yuan D, Chen P, Chen X, et al. A fiber supercapacitor with high energy density based on hollow graphene/conducting polymer fiber electrode. Adv Mater. 2016;28(19):3646-3652.
[DOI] -
138. Chen S, Ma W, Cheng Y, Weng Z, Sun B, Wang L, et al. Scalable non-liquid-crystal spinning of locally aligned graphene fibers for high-performance wearable supercapacitors. Nano Energy. 2015;15:642-653.
[DOI] -
139. Qiu H, Cheng H, Meng J, Wu G, Chen S. Magnetothermal microfluidic-assisted hierarchical microfibers for ultrahigh-energy-density supercapacitors. Angew Chem Int Ed. 2020;59(20):7934-7943.
[DOI] -
140. Han F, Jing W, Wu Q, Tian B, Lin Q, Wang C, et al. Nitrogen-doped graphene fiber electrodes with optimal micro-/meso-/macro-porosity ratios for high-performance flexible supercapacitors. J Power Sources. 2022;520:230866.
[DOI] -
141. Ma Y, Li P, Sedloff I, Zhang X, Zhang H, Liu J. Conductive graphene fibers for wire-shaped supercapacitors strengthened by unfunctionalized few-walled carbon nanotubes. ACS Nano. 2015;9(2):1352-1359.
[DOI] -
142. Ma W, Li W, Li M, Mao Q, Pan Z, Hu J, et al. Unzipped carbon nanotube/graphene hybrid fiber with less "dead volume" for ultrahigh volumetric energy density supercapacitors. Adv Funct Mater. 2021;31(19):2100195.
[DOI] -
143. Li Q, Cheng H, Wu X, Wang CF, Wu G, Chen S. Enriched carbon dots/graphene microfibers towards high-performance micro-supercapacitors. J Mater Chem A. 2018;6(29):14112-14119.
[DOI] -
144. Li Z, Lv W, Zhang C, Li B, Kang F, Yang QH. A sheet-like porous carbon for high-rate supercapacitors produced by the carbonization of an eggplant. Carbon. 2015;92:11-14.
[DOI] -
145. Jin Q, Li W, Wang K, Li H, Feng P, Zhang Z, et al. Tailoring 2D heteroatom-doped carbon nanosheets with dominated pseudocapacitive behaviors enabling fast and high-performance sodium storage. Adv Funct Mater. 2020;30(14):1909907.
[DOI] -
146. Shang T, Xu Y, Li P, Han J, Wu Z, Tao Y, et al. A bio-derived sheet-like porous carbon with thin-layer pore walls for ultrahigh-power supercapacitors. Nano Energy. 2020;70:104531.
[DOI] -
147. Wang C, Wu D, Wang H, Gao Z, Xu F, Jiang K. A green and scalable route to yield porous carbon sheets from biomass for supercapacitors with high capacity. J Mater Chem A. 2018;6(3):1244-1254.
[DOI] -
148. Afroze JD, Tong LY, Abden MJ, Chen Y. Multifunctional hierarchical graphene-carbon fiber hybrid aerogels for strain sensing and energy storage. Adv Compos Hybrid Mater. 2023;6:18.
[DOI] -
149. Zhang R, Yan J, Wang L, Shen W, Zhang J, Zhong M, et al. Achieving ion accessibility within graphene films by carbon nanofiber intercalation for high mass loading electrodes in supercapacitors. J Power Sources. 2021;513:230559.
[DOI] -
150. Wan S, Chen Y, Wang Y, Li G, Wang G, Liu L, et al. Ultrastrong graphene films via long-chain π-bridging. Matter. 2019;1(2):389-401.
[DOI] -
151. Fu Y, Xu L, Tian W, Liu Y, Cao D, Wang Q. Self-assembly of free-standing surface-oxidized multilayer graphene film for high volumetric supercapacitors. Carbon. 2023;213:118286.
[DOI] -
152. Lin Y, Plaza-Rivera CO, Hu L, Connell JW. Scalable dry-pressed electrodes based on holey graphene. Acc Chem Res. 2022;55(20):3020-3031.
[DOI] -
153. Guirguis A, Maina JW, Kong L, Henderson LC, Rana A, Li LH, et al. Perforation routes towards practical nano-porous graphene and analogous materials engineering. Carbon. 2019;155:660-673.
[DOI] -
154. Chen Z, An X, Dai L, Xu Y. Holey graphene-based nanocomposites for efficient electrochemical energy storage. Nano Energy. 2020;73:104762.
[DOI] -
155. Li Z, Xu Z, Liu YJ, Wang R, Gao C. Multifunctional non-woven fabrics of interfused graphene fibres. Nat Commun. 2016;7:13684.
[DOI] -
156. Li Z, Huang TQ, Gao W, Xu Z, Chang D, Zhang C, et al. Hydrothermally activated graphene fiber fabrics for textile electrodes of supercapacitors. ACS Nano. 2017;11(11):11056-11065.
[DOI] -
157. Shao F, Hu NT, Su YJ, Yao L, Li B, Zou C, et al. Non-woven fabric electrodes based on graphene-based fibers for areal-energy-dense flexible solid-state supercapacitors. Chem Eng J. 2020;392:123692.
[DOI] -
158. Guan T, Shen S, Cheng Z, Wu G, Bao N. Microfluidic-assembled hierarchical macro-microporous graphene fabrics towards high-performance robust supercapacitors. Chem Eng J. 2022;440:135878.
[DOI] -
159. Hou J, Jiang K, Wei R, Tahir M, Wu X, Shen M, et al. Popcorn-derived porous carbon flakes with an ultrahigh specific surface area for superior performance supercapacitors. ACS Appl Mater Interfaces. 2017;9(36):30626-30634.
[DOI] -
160. Pan L, Li X, Wang Y, Liu J, Tian W, Ning H, et al. 3D interconnected honeycomb-like and high rate performance porous carbons from petroleum asphalt for supercapacitors. Appl Surf Sci. 2018;444:739-746.
[DOI] -
161. Liu Y, Xiao Z, Liu Y, Fan LZ. Biowaste-derived 3D honeycomb-like porous carbon with binary-heteroatom doping for high-performance flexible solid-state supercapacitors. J Mater Chem A. 2018;6(1):160-166.
[DOI] -
162. Sun L, Zhou Y, Li L, Zhou H, Liu X, Zhang Q, et al. Facile and green synthesis of 3D honeycomb-like N/S-codoped hierarchically porous carbon materials from bio-protic salt for flexible, temperature-resistant supercapacitors. Appl Surf Sci. 2019;467-468:382-390.
[DOI] -
163. Sun Y, Xu D, He Z, Zhang Z, Fan L, Wang S. Green fabrication of pore-modulated carbon aerogels using a biological template for high-energy density supercapacitors. J Mater Chem A. 2023;11(37):20011-20020.
[DOI] -
164. Yang L, Wu D, Wang T, Jia D. B/N-codoped carbon nanosheets derived from the self-assembly of chitosan–amino acid gels for greatly improved supercapacitor performances. ACS Appl Mater Interfaces. 2020;12(16):18692-18704.
[DOI] -
165. Shi Y, Liu G, Jin R, Xu H, Wang Q, Gao S. Carbon materials from melamine sponges for supercapacitors and lithium battery electrode materials: A review. Carbon Energy. 2019;1(2):253-275.
[DOI] -
166. Ma M, Liu J, Huan Y, Ren M, Wei T, Yan S. Nitrogen-doped hierarchical nanoporous carbon materials from discarded polyurethane sponges via one-step double-activation method for double-layer capacitors. ACS Appl Nano Mater. 2024;7(3):2879-2888.
[DOI] -
167. Xiao K, Ding LX, Liu GX, Chen HB, Wang SQ. Freestanding, hydrophilic nitrogen-doped carbon foams for highly compressible all solid-state supercapacitors. Adv Mater. 2016;28(28):5997-6002.
[DOI] -
168. Xu J, Tan Z, Zeng W, Chen G, Wu S, Zhao Y, et al. Hierarchical carbon derived from sponge-templated activation of graphene oxide for high-performance supercapacitor electrodes. Adv Mater. 2016;28(26):5222-5228.
[DOI] -
169. Jing X, Wang L, Qu K, Li R, Kang W, Li H, et al. KOH chemical-activated porous carbon sponges for monolithic supercapacitor electrodes. ACS Appl Energy Mater. 2021;4(7):6768-6776.
[DOI] -
170. Peng H, Yao B, Wei X, Liu T, Kou T, Xiao P, et al. Pore and heteroatom engineered carbon foams for supercapacitors. Adv Energy Mater. 2019;9(19):1803665.
[DOI] -
171. Yao B, Peng H, Zhang H, Kang J, Zhu C, Delgado G, et al. Printing porous carbon aerogels for low temperature supercapacitors. Nano Lett. 2021;21(9):3731-3737.
[DOI] -
172. Wu X, Zhu X, Tao H, Wu G, Xu J, Bao N. Covalently aligned molybdenum disulfide-carbon nanotubes heteroarchitecture for high-performance electrochemical capacitors. Angew Chem Int Ed. 2021;60(39):21295-21303.
[DOI] -
173. Li C, Li X, Liu G, Yu W, Yang Z, Wang L, et al. Microcrack arrays in dense graphene films for fast-ion-diffusion supercapacitors. Small. 2023;19(33):2301533.
[DOI] -
174. Dong H, Zhang L, Liao Y, Huang K, Lian C, Zhou X, et al. Floating catalyst chemical vapor deposition patterning nitrogen-doped single-walled carbon nanotubes for shape tailorable and flexible micro-supercapacitors. Adv Funct Mater. 2023;33(29):2301103.
[DOI] -
175. Ren D, Zhang S, Dai J, Lan J, Qiu D, Zhang K, et al. Sulfur-functionalized carbon nanotubes with inlaid nanographene for 3D-printing micro-supercapacitors and a flexible self-powered sensing system. ACS Nano. 2024;18(31):20706-20715.
[DOI] -
176. Wu G, Tan P, Wu X, Peng L, Cheng H, Wang CF, et al. High-performance wearable micro-supercapacitors based on microfluidic-directed nitrogen-doped graphene fiber electrodes. Adv Funct Mater. 2017;27(36):1702493.
[DOI] -
177. Lee YG, Lee J, An GH. Surface engineering of carbon via coupled porosity tuning and heteroatom-doping for high-performance flexible fibrous supercapacitors. Adv Funct Mater. 2021;31(48):2104256.
[DOI] -
178. Qin T, Peng S, Hao J, Wen Y, Wang Z, Wang X, et al. Flexible and wearable all-solid-state supercapacitors with ultrahigh energy density based on a carbon fiber fabric electrode. Adv Energy Mater. 2017;7(20):1700409.
[DOI] -
179. Hu H, Yang C, Chen F, Li J, Jia X, Wang Y, et al. High-entropy engineering reinforced surface electronic states and structural defects of hierarchical metal oxides@graphene fibers toward high-performance wearable supercapacitors. Adv Mater. 2024;36(35):2406483.
[DOI] -
180. Gao L, Song J, Surjadi JU, Gao K, Han Y, Sun D, et al. Graphene-bridged multifunctional flexible fiber supercapacitor with high energy density. ACS Appl Mater Interfaces. 2018;10(34):28597-28607.
[DOI] -
181. Wang QF, Liu JH, Ran X, Zhang D, Shen G, Miao M. High-performance flexible self-powered strain sensor based on carbon nanotube/ZnSe/CoSe2 nanocomposite film electrodes. Nano Res. 2022;15:170-178.
[DOI] -
182. Park H, Song C, Jin SW, Lee H, Keum K, Lee YH, et al. High-performance flexible micro-supercapacitor for powering a vertically integrated skin-attachable strain sensor on a bio-inspired adhesive. Nano Energy. 2021;83:105837.
[DOI] -
183. Huang J, Gu J, Liu J, Guo J, Liu H, Hou K, et al. Environment-stable ionic organohydrogel as a self-powered integrated system for wearable electronics. J Mater Chem A. 2021;9(30):16345-16358.
[DOI] -
184. Wu T, Wu X, Li L, Hao M, Wu G, Zhang T, et al. Anisotropic boron-carbon hetero-nanosheets for ultrahigh energy density supercapacitors. Angew Chem Int Ed. 2020;59(52):23800-23289.
[DOI] -
185. Wang W, Xu L, Zhang L, Zhang A, Zhang J. Self-powered integrated sensing system with in-plane micro-supercapacitors for wearable electronics. Small. 2023;19(29):2207723.
[DOI] -
186. Manjakkal L, Nunez CG, Dang WT, Dahiya R. Flexible self-charging supercapacitor based on graphene-Ag-3D graphene foam electrodes. Nano Energy. 2018;51:604-612.
[DOI] -
187. Huang J, Peng S, Gu J, Chen G, Gao J, Zhang J, et al. Self-powered integrated system of a strain sensor and flexible all-solid-state supercapacitor using a high-performance ionic organohydrogel. Mater Horiz. 2020;7(8):2085-2096.
[DOI] -
188. He S, Hu Y, Wan J, Gao Q, Wang Y, Xie S, et al. Biocompatible carbon nanotube fibers for implantable supercapacitors. Carbon. 2017;122:162-167.
[DOI] -
189. Chae JS, Lee H, Kim SH, Chodankar NR, Kang SM, Lee S, et al. A durable high-energy implantable energy storage system with binder-free electrodes usable in body fluids. J Mater Chem A. 2022;10(9):4611-4620.
[DOI] -
190. Jang Y, Park T, Kim E, Park JW, Lee DY, Kim SJ. Implantable biosupercapacitor inspired by the cellular redox system. Angew Chem Int Ed. 2021;60(19):10563-10567.
[DOI] -
191. Wang X, Yu M, Kamal HM, Niu J, Zhang Y, Zhou Q, et al. An anticoagulant supercapacitor for implantable applications. Nat Commun. 2024;15:10497.
[DOI] -
192. Hu M, Wang J, Liu J, Wang P, Feng Y, Wang H, et al. A flour-based one-stop supercapacitor with intrinsic self-healability and stretchability after self-healing and biodegradability. Energy Storage Mater. 2019;21:174-179.
[DOI] -
193. Huang S, Zhu X, Sarkar S, Zhao Y. Challenges and opportunities for supercapacitors. APL Mater. 2019;7:100901.
[DOI] -
194. Yang Y, Han Y, Jiang W, Zhang Y, Xu Y, Ahmed AMA. Application of the Supercapacitor for Energy Storage in China: Role and Strategy. Appl Sci. 2022;12(1):354.
[DOI]
Copyright
© The Author(s) 2025. This is an Open Access article licensed under a Creative Commons Attribution 4.0 International License (https://creativecommons.org/licenses/by/4.0/), which permits unrestricted use, sharing, adaptation, distribution and reproduction in any medium or format, for any purpose, even commercially, as long as you give appropriate credit to the original author(s) and the source, provide a link to the Creative Commons license, and indicate if changes were made.
Publisher’s Note
Share And Cite