Abstract
High-performance and long-lifetime blue organic light-emitting diodes (OLEDs) are crucial for meeting the demands of advanced display and lighting technologies. Despite high device efficiency has been achieved in blue OLEDs, development of high-performance and long-lifetime blue OLEDs still lag far behind their red/green counterparts due to the presence of long-lived high-energy triplet excitons and polarons. Given the critical role of charge and exciton management in both the emission and degradation processes of OLEDs, this review systematically summarizes strategies for suppressing charge leakage and exciton quenching, as well as for enhancing exciton utilization in blue fluorescent, phosphorescent, and thermally activated delayed fluorescent (TADF) OLEDs. In this context, we further discuss the roles of conventional fluorescent hosts, triplet-triplet annihilation/hot exciton hosts, TADF assistant hosts, phosphorescent assistant hosts, and exciplex/electroplex hosts in regulating charge and exciton dynamics in blue OLEDs. Additionally, the modification of emitting layer materials is highlighted as a key strategy for managing charge and exciton processes in efficient and stable solution-processed blue OLEDs. Based on current insights into the efficiency and operational stability of blue OLEDs, this review proposes feasible charge and exciton management strategies to address the current challenges.
Keywords
1. Introduction
Recently, organic light-emitting diodes (OLEDs) have emerged as a mainstream technology in large-area display and general lighting markets owing to their outstanding properties such as high brightness, wide color gamut, low power consumption, fast response time and environmental friendliness[1-3]. To fully meet the commercial standards of high-quality display and lighting applications, the development of high-efficiency and long-lifetime OLEDs is particularly critical, ensuring superior electro-optical conversion efficiency and enhanced operational stability[2,4]. Since the first generation of fluorescent materials (Alq3) was reported in 1987, the immense potential of organic materials in electroluminescent has been widely recognized[5]. However, due to their low exciton utilization efficiency (EUE), fluorescent emitters have faced limitations in device efficiency, promoting the development of more advanced emitters, such as phosphorescence and thermally activated delayed fluorescence (TADF) materials, which can harvest both singlet and triplet excitons to achieve 100% internal quantum efficiency (IQE). To date, red and green phosphorescent emitters have been successfully implemented in commercialized OLED production. However, the poor operational stability of blue OLEDs remains a significant hurdle. As a result, current industrial OLED displays still rely on traditional blue fluorescent emitters, which suffer from relatively low device efficiency. Thereby, developing high-performance, long-lifetime blue OLEDs is an urgent and ongoing challenge in the field.
In terms of blue OLED devices, achieving high external quantum efficiency (EQE) heavily relies on the efficient utilization of triplet excitons. However, triplet excitons typically possess long excited state lifetimes, and their prolonged residence time within the device increases the possibility of triplet exciton collision. When two triplet excitons collide, their interaction can result in an energy state approximately double that of a single triplet, generating high-energy species that are likely to induce irreversible dissociation of weak chemical bond in the emitting materials. This issue is particularly severe for blue emitters, which possess higher triplet energy levels compared to red and green emitters, making them more vulnerable to excition-induced degradation[6-9]. So, bond dissociation energy (BDE) is a critical parameter for quantitatively assessing the intrinsic stability of organic materials under device operation. A higher BDE value implies that more energy is required to break the molecular bonds, suggesting greater photochemical and electrochemical stability[10]. Thereby, the design and synthesis of blue-emitting materials with robust chemical bonds are essential for ensuring long-term device performance and operational durability. When carriers are injected from the electrodes and transported to the emission layer (EML) through the transport functional layers, they recombine through Coulombic interactions to form excitons, which subsequently undergo radiative transition to emit light. However, imbalances in charge transport often result in excess holes or electrons, which are then carried by neutral molecules. These excess charges generate positive or negative polarons, and their interactions with triplet excitons (namely triplet-polaron annihilation, TPA) further accelerate device degradation. Meanwhile, high triplet exciton densities tend to accumulate near the interface with poor charge transport, exacerbating both triplet-triplet annihilation (TTA) and TPA processes. More importantly, when electrons and holes leak into adjacent transporting layers, the hole transport layer (HTL) becomes cationic, and the electron transport layer (ETL) becomes anionic. These ionized states are more susceptible to bond dissociation, especially upon receiving energy transfer from the neighboring EML. The degradation of transport layer molecular further aggravate the imbalance in charge transporting, ultimately resulting in a shorter device lifetime[11-13]. In addition, the decomposition of organic materials generates free radical fragments and further react with the neighboring molecules to form lower energy by-products, subsequently evolved into defects act as exciton quenchers, deep charge traps and non-radiative recombination centers to further age the devices. Thereby, a comprehensive understanding of blue OLEDs degradation mechanisms, along with strategies to improve charge transport balance and optimize exciton dynamics, is crucial for achieving high-performance and durable blue OLEDs.
In a brief summary, the short operational lifetime of blue OLEDs remains a major bottleneck in commercialization growth. In this contribution, charge and exciton management strategies for achieving high-performance and long-lifetime blue OLEDs are categorized and elaborated, the significant success in their device performance reveal exciton allocation and charge transporting play a crucial role in the balance of efficiency and stability. Finally, based on the consideration of many aspects, the possible charge and exciton management strategies are proposed and discussed for high-performance blue OLEDs satisfying the commercialized standards.
2. Basical Standards of Blue OLEDs
2.1 Turn-on voltage (Von)
The Von of an OLED device is typically defined as the voltage of device at a luminance of 1 cd/m2. Von has a significant influence in device performance, such as the lower Von facilitates reducing the power consumption in OLED display and lighting applications. Generally, improved ohmic contact between the electrodes and organic functional layers can reduce the barrier for electron and hole injection, and thus a lower Von for device. Additionally, efficient charge injection and transport across the organic functional layers is essential for achieving a low Von but also for ensuring high brightness and enhanced device efficiency.
2.2 Luminance
Luminance refers to the luminous intensity emitted by a light source per unit area, measured in cd/m2.
2.3 Luminous efficiency
2.3.1 EQE
EQE represents the ratio of the total number of photons emitted from the device to the number of injected charge carriers. It is a critical parameter for evaluating the electroluminescent performance of blue OLEDs, directly reflecting the efficiency of charge-to-light energy conversion. EQE can be expressed as:
where ηout is the light out-coupling efficiency, primarily determined by the refractive index differences between the functional layers within the device, which typically ranges from 0.2 to 0.3; ΦPL is the photoluminescence quantum yield of the emitter; γ is the charge balance factor, dictated by the balance of electron and hole transport within the device; ηST is the radiative exciton fraction, defined as the ratio of excitons that undergo radiative transitions. Furthermore, the maximum EQE (EQEmax) is commonly used to evaluate device performance.
2.3.2 Current efficiency (CE)
CE is the ratio of luminance to current density, expressed in units of cd/A, where higher current efficiency indicates that a device can produce higher luminance at lower current levels. Additionally, in blue OLED devices, the ratio of CE to CIEy, termed CCE, is used to evaluate both the efficiency and color purity of the blue emission.
2.3.3 Power efficiency (PE)
PE is the ratio of luminous output power to electrical input power, measured in lm/W. A higher power efficiency indicates that more light is generated for the same amount of power, which reflects better energy conversion efficiency of the device.
2.3.4 Roll off
The efficiency roll-off in OLED refers to a significant decrease in EQE or luminous efficiency with increasing current density or luminance.
2.4 CIEy
The CIEy value is a chromaticity coordinate in the Commission Internationale de l'Eclairage (CIE) 1931 color space, primarily representing the green component of a color. A lower CIEy value indicates reduced green content, signifying purer blue emission. Therefore, the CIEy value is crucial for defining the precise shade of blue. Specifically, CIEy values below 0.05, 0.10, 0.25, and 0.35 correspond to ultra-deep blue, deep blue, blue, and sky blue, respectively[2]. Different OLEDs applications have varying CIEy requirements. In high-definition display applications, a lower CIEy value can provide a higher color gamut coverage and deeper blacks, and thus a greater color saturation and contrast. Conversely, blue with a higher CIEy value is suitable for general lighting applications due to its higher brightness and color uniformity. Notably, the color purity of blue light also affects the electroluminescent performance of OLEDs. As shifting deep blue to sky blue region, the luminous efficacy of radiation for white light drops significantly, leading to higher power consumption in OLED-based applications[14].
2.5 Operational lifetime
The operational lifetime of OLED devices (LTX) is typically defined as the time required for the initial brightness declines to its X% under constant current driving. Given the sensitivity of human eye to brightness decay of approximately 3%-5%, LT97 and LT95 are commonly used as a commercialized standard of OLED device stability.
2.6 Blue spectrum and full width at half-maximum (FWHM)
The spectrum represents the distribution of a light source's energy across different wavelengths, with blue light typically falling within the 400-500 nm range. The spectral distribution determines the color purity and color gamut of blue OLEDs. FWHM refers to the full width at half of its maximum spectral intensity, which reflects the sharpness of the spectrum, a smaller FWHM signifies a narrower spectrum and a higher color purity.
3. Charge/Exciton Managing Strategies for High-Performance and Long-Lifetime Blue OLEDs
Due to the different emission mechanisms of luminescent materials, the charge and exciton management strategies for improving device efficiency and lifetime of blue OLEDs are not exactly the same. The emission mechanisms of various blue emitters are shown in Figure 1. Based on these mechanisms, charge and exciton management strategies for high-performance and long-lifetime blue OLEDs are mainly divided into four categories, including blue fluorescent OLEDs, blue phosphorescent OLEDs, blue TADF OLEDs and host engineering for high-performance and long-lifetime blue OLEDs. Additionally, the operational stability progress of solution-processed blue OLEDs is also briefly introduced.
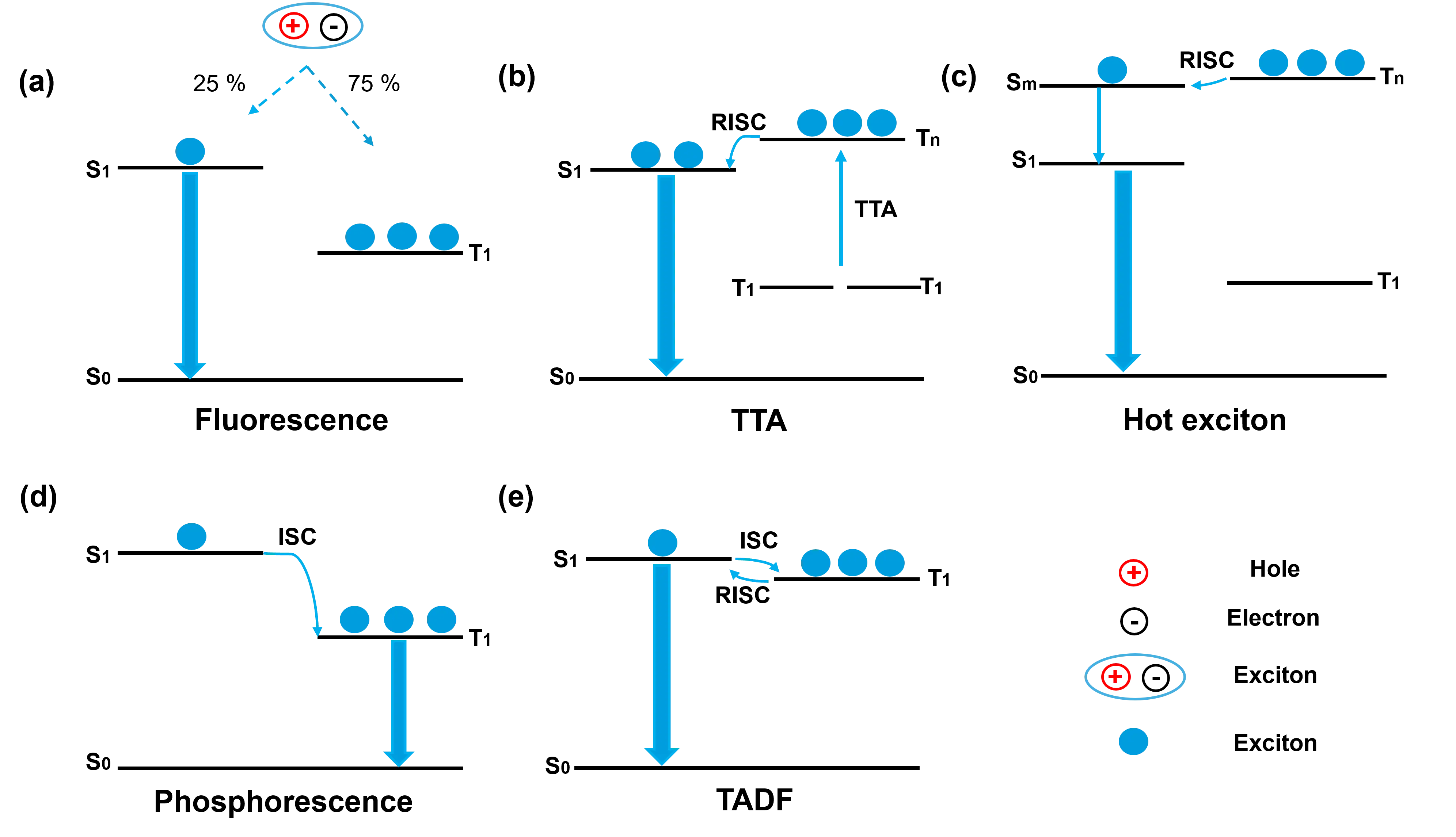
Figure 1. The emission mechanisms of (a) Fluorescent emitters; (b) TTA emitters; (c) hot exciton emitters; (d) phosphorescent emitters; (e) TADF emitters. TTA: triplet-triplet annihilation; TADF: thermally activated delayed fluorescence; RISC: reverse intersystem crossing.
3.1 Blue fluorescent OLEDs
3.1.1 Blue OLEDs based on traditional fluorescent emitters
Compared with blue phosphorescent and TADF emitters, traditional blue fluorescent emitters offer better color purity and durability, making them widely used in the current display and lighting applications, although low device efficiency for the developed fluorescent OLEDs[15,16]. Fortunately, significant efforts have been devoted to improving the efficiency and operational lifetime of blue fluorescent OLEDs.
As mentioned above, charge leakage is a critical factor impacting the operational lifetime of blue OLEDs. Advanced device designs can effectively suppress the degradation of the transport layer materials caused by charge leakage. In 2023, Song et al. improved the lifetime of blue fluorescent OLEDs by introducing electron leakage pathways (ELP) in the electron blocking layer (EBL), which helped reduce the formation of unstable anionic states in the EBL[13]. They employed two TTA materials, 9-(3(phenanthrene-9yl)phenyl)-10-phenylanthracene (DPAP) and 10-(dibenzo[b,d]furan-4-yl)-9-phenylanthracene, which were used as ELP materials doped in EBL and as hosts for a blue fluorescent emitter BD. As shown in Figure 2a, the ELP material doped in the EBL has a lowest unoccupied molecular orbital (LUMO) level similar to that of the host material in the EML, allowing electrons to flow into the ELP material without overcoming an energy barrier, rather than leaking from the EML into the EBL. DPAP, with strong BDE in anionic state, was selected as the ELP materials, effectively suppressing degradation of both the EBL and ELP materials in anionic states. Therefore, the device based on DPAP achieved a LT90 of 400 hours at 1,000 cd/m2, which demonstrated a 33% improvement in lifetime compared to the devices without the ELP, simultaneously with an EQEmax of 9.44%, as shown in Figure 2b,c.
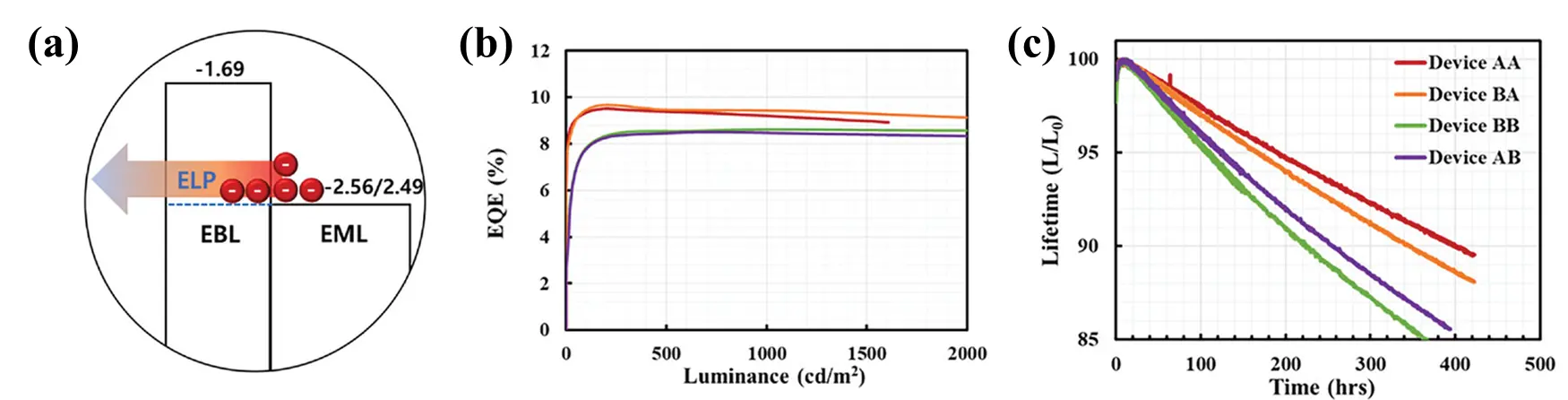
Figure 2. (a) Schematic diagram of ELP mechanism; (b) EQEs of the devices with or without ELP; (c) device lifetimes measured under the constant current density of the initial luminance of 1,000 cd/m2. Republished with permission from[13]. ELP: electron leakage pathways; EBL: electron blocking layer; EML: emission layer; EQE: external quantum efficiency.
However, for blue fluorescent OLEDs, once stability standards are met, achieving high exciton utilization should become the primary target. Improving the luminescent properties of blue fluorescent emitters can further enhance the performance of blue fluorescent OLEDs. In 2023, Bai et al. designed and synthesized three boron-based blue emitters with asymmetric structures by combining a tetrahydroquinoline type donor, a boron core, and an additional pairing donor[17]. These include B-N-S-1 and B-N-S-2 with TADF characteristics and the fluorescent emitter B-N-S-3. Notably, the fluorescent emitter B-N-S-3, which exhibited the lowest intersystem crossing rate (kISC), demonstrated superior performance in blue OLED based on the TTA host ANDN. The device achieved an EQEmax of 6.7%, a LT95 of 136 hours at 1,000 cd/m2, with a peak emission at 461 nm and a narrow FWHM of 35 nm. The kISC was identified as a critical factor influencing the device's performance. Specifically, a higher kISC leads to more triplet excitons being generated in the emitter, which are subsequently quenched by the host with the lower first triplet state (T1) energy, and thus severe energy loss. Additionally, the presence of these high-energy triplet excitons in the EML accelerates the degradation of EML materials. Therefore, reducing the kISC of fluorescent emitters is a viable strategy to improve the performance of blue fluorescent OLED.
However, the limited exciton utilization of blue fluorescent emitters hinders blue fluorescent OLEDs from achieving high efficiency. Fortunately, assistant host strategies for blue fluorescent OLEDs have demonstrated significant potential in achieving highly efficient and long-lifetime blue OLEDs (as discussed in detail in Section 3.4)[18-20]. Moreover, developing stable blue fluorescent emitters with higher exciton utilization could offer more possibilities for precise exciton management.
3.1.2 Blue OLEDs based on TTA emitters
TTA-type fluorescent emitters can achieve higher IQE (up to 62.5%) by undergoing the collision of two low-energy triplet excitons to generate additional singlet excitons[21-23]. Notably, an ideal TTA emitter should has a singlet excited-state energy lower than the energy of triplet excited state pair, and the energy of the triplet excited state pair should be lower than that of the second and higher triplet states (Tn, n ≥ 2)[23].
For blue TTA emitters, a narrow recombination region facilitates increasing triplet exciton density to promote TTA process, and thus the improved efficiency for OLEDs[24,25]. Generally, a narrow recombination zone is likely to form at the interface between the EML and the adjacent layers. In 2022, Nguyen et al. demonstrated that interfacial charge transfer (CT) between EBL and EML can be beneficial to the performance of pure blue TTA-OLEDs[26]. A small energy offset at the interface promotes the formation of exciplexes between the EBL (Tris-PCz) and the TTA emitter (NaNaP-A) in the EML. Subsequently, the high-density interfacial triplet excitons (3CT) were rapidly quenched by the low triplet energy of NaNaP-A (3LE), generating a high density of 3LE excitons, which effectively facilitated the TTA process. Furthermore, the close energy levels between the singlet of NaNaP-A (1LE) and the singlet CT (1CT) enable both the interfacial exciplex and the TTA emitter to contribute to fluorescence emission. Thus, a blue OLED with interfacial CT exciton-sensitized TTA emitters achieved an EQEmax of 6.2%, which is more than three times that of devices without interfacial CT interaction. Meanwhile, the electron and hole were used to form the interfacial exciplex reduced the holes injected into the EML, significantly lowering the Von of device. To further enhance device performance and improve color purity, the blue emitter v-DABNA with a suitable the lowest singlet excited state (S1) energy, was introduced into the interface CT sensitized TTA blue OLED. The energy from 1CT (interface) and 1LE (NaNaP-A) can be completely transferred to v-DABNA, resulting in the device achieving a high EQEmax of 8% and pure blue emission with CIE coordinates of (0.12, 0.12). Moreover, the blue TTA-OLED based on the TPN-DBF/NaNaP-A interfacial exciplex also exhibited excellent device performance, achieving an EQEmax of 10% and a LT95 of over 1,000 hours at 1,000 cd/m2[27].
However, most TTA emitters exhibit relatively low photoluminescence quantum yield (PLQY), and the quenching processes of triplet excitons within the EML are difficult to mitigate[28]. As a result, OLEDs based on non-doped TTA emitters suffer from low up-conversion efficiency, leading low device efficiency (less than 12.5%). Fortunately, TTA materials used as hosts doped with fluorescent emitters can exhibit good device performance. With the implementation of additional charge and exciton management strategies, blue OLEDs meet the commercial standards can be realized, as discussed in Section 3.4.2.
3.1.3 Blue OLEDs based on hot exciton emitters
Besides, hot exciton materials can achieve 100% triplet exciton utilization by exploiting the high-lying reverse intersystem crossing (hRISC) process, which transfers triplet excitons from high-lying triplet states (Tn ≥ 2) to singlet excitons[29-32]. An ideal hot exciton material generally possesses a large Tn - T1 energy gap, which suppresses the internal conversion (IC) process from Tn to T1, while a small Tn - Sm energy splitting (n ≥ 2, m ≥ 1) facilitates efficient reverse intersystem crossing (RISC) process to harvest triplet excitons[33].
The RISC process is essential for achieving high performance in blue OLEDs based on hot exciton emitters. Recent studies therefore focus on designing hot exciton emitters with additional triplet energy levels close to S1, which opens more hRISC channels and accelerates triplet exciton utilization. In 2022, Yu et al. developed a pyrene-core-based blue hot exciton emitter (CPPCN) featuring multiple hRISC pathways[34]. CPPCN has two high-lying triplet states (T2 and T3) close to S1, with small energy differences of 0.02 eV and 0.15 eV, respectively, which facilitate the RISC process from the high-lying triplet states to the singlet state, thereby increasing triplet exciton utilization. Additionally, CPPCN exhibits a high hRISC rate (k hRISC) of 2.86 × 108 s-1, which reduces the accumulation of triplet excitons. The fabricated non-doped device with an architecture of ITO/PEDOT: PSS/TCTA/CPPCN/TPBi/LiF/Al, achieved a high EQEmax of 11.64%, a CIEy value of 0.17 and low efficiency roll-off at high brightness (EQE above 8.5% at 10,000 cd/m2). Moreover, the device using non-doped CPPCN as the EML demonstrated an estimated LT95 of 368 hours at 1,000 cd/m2, revealing the significant potential in achieving high-performance and long-lifetime blue OLEDs by using pyrene-based blue hot exciton materials with multiple hRISC pathways.
In 2023, Du et al. designed and synthesized a blue hot exciton emitter PPITPh by combining the phenanthro[9,10-d]imidazole (PI) moiety and m-terphenyl group via benzene bridge[35]. Due to the small energy gaps between T2/T3 and S1 (0.23 eV and 0.09 eV, respectively), PPITPh efficiently harvests triplet excitons through two hRISC channels (with khRISC of 1.18 × 107 s-1 and 1.55 × 107 s-1, respectively). As a result, the non-doped PPITPh-based blue OLED achieved a high EQE of 11.83%, low efficiency roll-off (with an EQE of 7.50% at 10,000 cd/m2), and deep blue emission with CIEy of 0.07. Additionally, the LT50 at 100 cd/m2 was 48.4 hours.
Additionally, in 2024, Xu et al. designed and synthesized a blue hot exciton emitter, o-CPPCN, by modifying the donor linking type of CPPCN to an ortho-substituted, resulting in deeper blue emission (448 nm) and a higher khRISC (3.1 × 108 s -1)[36]. Under the same device architecture, the non-doped o-CPPCN-based blue OLED achieved a high EQE of 10.3% and superior color purity (CIEy of 0.11). The high kRISC rate enabled o-CPPCN to achieve a high EUE of 55%, which is significantly higher than the 44% of CPPCN. Unfortunately, the poor charge transport balance contributes to the severe efficiency roll-off (Roll-off of 51% at 1,000 cd/m2).
In short, low device efficiency remains a significant bottleneck for traditional fluorescent emitters in blue OLEDs. TTA/hot exciton type blue fluorescent emitters with improved EUE and favorable stability are promising candidates for commercialization, but it remains to be seen. The chemical structures of traditional fluorescent emitters, TTA emitters and hot exciton emitters are shown in Figure 3.
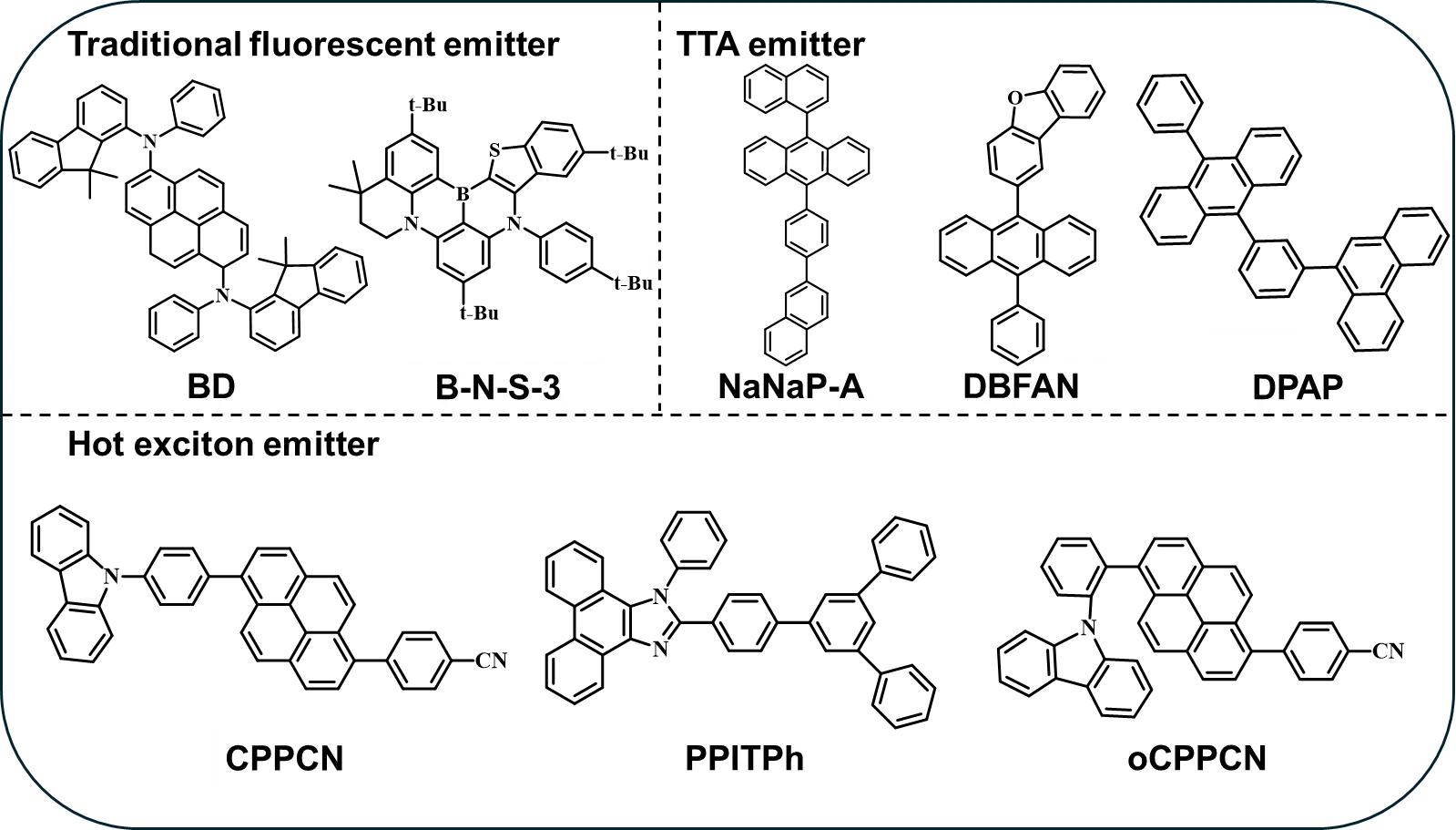
Figure 3. Chemical structures of traditional fluorescent emitters, TTA emitters and hot exciton emitters. TTA: triplet-triplet annihilation.
3.2 Blue phosphorescent OLEDs
Blue phosphorescent emitters containing heavy metal atom have been developed to harvest non-radiative triplet excitons, achieving high efficiency in blue OLEDs[37-39]. Unfortunately, the slow radiative transition of the T1 exciton state results in a prolonged triplet exciton lifetime, which significantly affects the diffusion behavior of triplet excitons. Particularly under unbalanced charge transport conditions, triplet excitons are prone to diffuse towards the boundaries of the EML, leading to exciton leakage. Furthermore, the accumulated long-lived triplet excitons within the EML, subsequently triggering unfavorable bimolecular processes, such as TTA and TPA interactions. The generated high-energy excitons and polaron can easily break the inherently weak metal-ligand bonds of blue phosphorescent emitters, thereby leading to the reduced operational lifetime of blue phosphorescent OLEDs[40,41]. Thus, strategies that extend the lifetime of blue phosphorescent devices without compromising efficiency hold significant potential for high-performance blue OLEDs.
Charges and excitons leaking from the EML into adjacent layers not only results in efficiency loss but also degrade the adjacent layer materials, leading to shortened device lifetime. Inserting an EBL layer with appropriate charge transport properties and high triplet energy level between the EML and transport layer allows for balanced charge transport while confining triplet excitons within the EML. Therefore, this serves as an efficient strategy to prevent triplet exciton leakage caused by diffusion and charge leakage. In 2015, Seo et al. designed and synthesized a stable, high triplet energy EBL material 9,9-dimethyl-10-(9-phenyl-9H-carbazol-3-yl)-9,10-dihydroacridine (PCZAC)[42]. Benefiting from the high triplet energy levels, devices using 3,3'-di(9H-carbazol-9-yl)-1,1'-biphenyl (mCBP) and PCZAC as EBLs effectively prevent the quenching of the triplet excitons of the emitter tris[1-(2,4-diisopropyldibenzo[b,d]furan-3-yl)-2-phenylimidazole] iridium(III) (Ir(dbi)3). In addition, the hole-trapping characteristics of the blue phosphorescent emitter tend to form exciton near the interface of hole-transport layer (HTL) and EML. Compared with devices using mCBP as EBL, PCZAC has a more compatible highest occupied molecular orbital (HOMO) level with the HTL and host, offers a lower hole injection barrier, which allows more holes to be injected into the EML for harvesting triplet excitons, thereby improving device efficiency. Furthermore, PCZAC with superior hole injection and transport properties, significantly reduces charge accumulation, thus enhancing the stability of blue phosphorescence devices. As a result, an EQEmax of nearly 14% and a LT70 of 180 hours at 500 cd/m2 (eight times) were achieved for device based on PCZAC.
Furthermore, they proposed an alternative strategy. In 2016, they introduced the green phosphorescent emitter iridium (III) bis(2-phenylpyridinato-N,C20)acetylacetonate (Ir(ppy)2(acac)) into the ETL to harvest blue phosphorescent triplet excitons diffusing from the EML[43]. The energy transfer channel from the diffused blue phosphorescent triplet excitons to the green phosphorescent triplet emitter improved the efficiency of the blue phosphorescent OLED. Moreover, the energy transfer process prevented the quenching of blue phosphorescent triplet excitons by the triplet excitons of the ETL, inhibiting ETL degradation and doubling the lifetime of the blue phosphorescent OLED based on emitter Ir(dbi)3. As a result, an EQEmax of 11.2%, low roll-off (The EQE at 1,000 cd/m2 is 8.8%) and a LT70 of 47 hours at 1,000 cd/m2 were realized for the blue phosphorescent OLED. However, only partial exciton utilization contributes to blue emission, while weak green emission impacts the CIEy of blue phosphorescent OLEDs, thereby affecting blue purity.
In addition to inserting blocking layers, modulating charge transport balance through the charge-capturing capacity of the emitter to expand the recombination zone and ensure exciton utilization within the EML is considered an alternative strategy for high-performance blue phosphorescent OLEDs. In 2014, Zhang et al. demonstrated a blue phosphorescent OLED with a graded dopant concentration profile in a broadened EML[44]. In this device, holes are transported through the blue phosphorescent emitter iridium (III) tris[3-(2,6-dimethylphenyl)-7-methylimidazo[1,2-f] phenanthridine] (Ir(dmp)3), with a gradually decreasing doping concentration of the blue phosphorescent emitter from the anode to the cathode. The device structure with a graded doping concentration broadens the distribution of excitons within the EML, significantly reducing the overall exciton density and thereby suppressing the TPA degradation. Consequently, the device with graded dopant concentrations achieved an EQE of 9.5% and a LT80 of 213 hours at 1,000 cd/m2. Additionally, when this strategy was applied to fabricate a stacked PHOLED, a high EQE of 18% and a LT80 of 616 hours at 1,000 cd/m2 were achieved, which is more than double the efficiency and over ten times the lifetime of traditional blue phosphorescent devices.
Furthermore, in 2019, Klimes et al. proposed a device structure featuring step-wise graded doping of the blue phosphorescent emitter PtNON[45]. The EML is divided into three sections based on the doping concentration of the blue emitter, which enhances charge transport balance and expands the exciton recombination zone. In addition, the linear graded- doping strategy shifted the charge recombination zone away from the EBL, effectively preventing exciton quenching by the low triplet energy level of the EBL and thereby improving device performance. Therefore, the blue phosphorescent OLED based on the linear graded-doping strategy exhibited a high EQEmax of 17.4% (The EQE is of 17.4% at 1,000 cd/m2) and an estimated LT70 of 1,337 hours at 1,000 cd/m2.
Additionally, in 2019, Han et al. developed a double-emitting-layer (d-EML) blue phosphorescent OLED by using two blue phosphorescence materials, fac-tris(3-(1-(2,6-diisopropylphenyl)-1H-imidazole-2-yl)benzonitrile) (CNImIr) and fac-tris(3-(2,6-diisobutylphenyl)imidazo[1,2-f]phenanthridine)iridium (iPrImIr), both of which have similar emission spectra. Both emitters exhibit electron-trapping and hole-trapping properties, respectively[46]. The proposed d-EML structure utilizes the carrier-trapping properties of phosphorescent emitters to confine the exciton recombination zone and achieve balanced charge transport, thereby preventing the leakage of charges and triplet excitons. Accordingly, a sixfold increase in the lifetime of d-EML blue phosphorescent OLED compared with single emitting layer device was achieved, with a higher EQEmax of 16.1% and a LT95 of 13.8 hours at 1,000 cd/m2.
However, achieving charge transport balance through carrier trapping within the EML is likely to trigger TPA interaction, which is detrimental to the lifetime of blue phosphorescent OLEDs. In 2016, Zhang et al. investigated the degradation mechanisms of blue phosphorescent OLED under exciton-polaron interactions[47]. The results revealed that the primary cause for device degradation originated from TPA process, which caused the reduction of quantum yield of EML, the secondary cause was charge imbalance induced by the generated charge traps during the TPA process. Therefore, in addition to preventing exciton leakage, it is essential to achieve charge transport balance within the EML to avoid the generation of excess carriers.
Transferring the charge-trapping sites from the EML to the transport layers can effectively reduce polaron formation within the EML while regulating balanced charge transport. In 2017, Song et al. doped the blue phosphorescent emitter tris[2-(4-fluorophenyl)-1-(50-isopropyl-[1,10:30,100-terphenyl]-20-yl)-1Himidazole] (Ir(fim)3) into HTL to reduce additional positive polarons formation within EML through weak hole trapping effect, and thus decreasing the interaction between the triplet exciton and positive polaron. This is conductive to extending the lifetime of blue phosphorescent OLED based on the terminal emitter Ir(dbi)3[48]. The optimized device structure exhibited double the device lifetime compared with the non-doped HTL devices, with a high EQEmax of 13% and a LT70 of 118 hours at 1,000 cd/m2.
The trapping behavior of the emitter can be modulated by reducing molecular orbital gap between the host and emitter, thereby reducing polarons. In 2017, Song et al. investigated the aging mechanisms of single-carrier devices with bis[2-(4,6-difluorophenyl)pyridinato-C2,N](picolinato) (FIrpic) doped into m-CBP host as the EML under exciton, polaron, and exciton-polaron interactions[49]. The larger LUMO gap between FIrpic and the m-CBP host increased electron trapping by FIrpic, making the device more susceptible to TPA degradation. Replacing mCBP with a bipolar host CzTrz that has a more compatible LUMO level, which can effectively suppress electron trapping by FIrpic and TPA degradation under negative polaron conditions, and thus extending the lifetime of the blue OLED based on CzTrz (three times). Further details on the suppression of the TPA process induced by carrier trapping are discussed extensively in Section 3.4.
Although balanced charge transport reduces charge leakage and promotes uniform exciton distribution within the EML, TTA and TPA processes remain unavoidable. Thus, mitigating the impact of TTA and TPA on the device can also help sustain the performance of blue phosphorescent OLEDs. In 2017, Lee et al. introduced a hot excited state manager meridional-tris-(N-phenyl, N-methyl-pyridoimidazol-2-yl)iridium (III) (mer-Ir(pmp)3) into the EML[40]. This facilitates exothermic energy transfer from the hot excited state to the manager, thereby preventing the dissociation of the phosphorescent emitter Ir(dmp)3 or the host m-CBP. Additionally, the triplet energy of the hot excited state manager is higher than that of the phosphorescent emitter, allowing the efficient energy transfer process from the manager to the emitter. Notably, introducing the manager into the EML region with the highest triplet exciton density, where bimolecular annihilation processes are most likely to happen, can effectively suppress device degradation. Thus, the device achieved a LT80 of 334 hours at 1,000 cd/m2, which was 3.6 times longer than the devices without the manager, simultaneously with a high EQEmax of 9.6%.
In summary, managing the leakage of charges and excitons from the EML and broadening the exciton recombination zone are crucial for high-performance and long-lifetime blue phosphorescent OLEDs. The chemical structures of phosphorescent emitters are shown in Figure 4.
3.3 Blue thermally activated delayed fluorescent OLEDs
Purely organic TADF emitters with a small ΔEST can harvest triplet excitons for radiative transitions by taking advantage of RISC process from triplet states to singlet states. However, the longer triplet exciton lifetime in TADF OLEDs is vulnerable to more severe triplet-exciton-related degradation reactions compared to phosphorescent OLEDs[10]. Additionally, TADF materials typically have a small ΔEST due to their separated HOMO and LUMO distributions through the connection of donor and acceptor groups. The resulting CT emission broadens its bandwidth, hindering its application in high-definition displays that require narrow-band emission[50,51]. Fortunately, Hatakeyama et al. achieved narrow-band blue TADF emission by utilizing multiple resonance (MR) effects to suppress structural relaxation and vibrational coupling to give high color purity and PLQY[50-52]. However, the slower RISC process and the longer triplet exciton lifetime significantly inhibit the operational lifetime of blue MR-TADF OLEDs. Similarly, charge and exciton management strategies aimed at extending the lifetime of blue TADF-OLEDs can foster the development of high-performance, long-lifetime blue OLEDs.
Similar to blue phosphorescent OLEDs, achieving balanced charge transport and reducing charge and exciton leakage can be effectively accomplished by applying transport/blocking layers that possess high triplet energy levels, stability, and excellent charge transport properties. In 2020, Kamata et al. developed a multifunctional HTL material, 4DBFHPB, to address efficiency and lifetime issues in blue TADF-OLEDs[53]. 4DBFHPB with excellent hole transport property and anion stability, minimizes the formation of anion-state 4DBFHPB and reduces anion-induced device degradation. Additionally, its deep ionization potential and high triplet energy inhibits the formation of exciplexes between the HTL and the TADF emitter to prevent the quenching of triplet excitons. Therefore, a sky-blue TADF-OLED based on the 2,3,4,5,6-pentakis(3,6-di-tert-butyl-9H-carbazol-9-yl)benzonitrile (5TCzBN) emitter and 4DBFHPB HTL achieved a high EQEmax of 21.5% (The EQE is of 17.3% at 1,000 cd/m2) and an estimated LT50 of 1,700 hours at 500 cd/m2.
In 2023, Jiang et al. designed and synthesized two spiro core-based hole-transporting materials (HTMs) N-([1,1'-biphenyl]-4-yl)-N-(9,9-dimethyl-9H-fluoren-2-yl)spiro[fluorene-9,9′-xanthen]-2-amine (FBP-SFX) and N-([1,1'-biphenyl]-4-yl)-N-(dibenzo[b,d]furan-4-yl)spiro[fluorene-9,9'-xanthen]-2-amine (DBF-SFX) featuring deep HOMO levels and strong BDE[54]. The device based on MR-TADF emitter 2,12-di-tert-butyl-5,9-bis(4-(tert-butyl)phenyl)-7-methyl-5,9-dihydro-5,9-diaza-13b-boranaphtho[3,2,1-de]anthracene (M-t-DABNA) and HTM DBF-SFX achieved a high EQEmax of 33.2%, nearly three times that of the devices based on NPB and FBP-SFX HTMs, which arose from its larger bandgap, deeper HOMO level, lower trap density and balanced charge carrier mobility. Additionally, to weaken the impact of the long-lifetime triplet exciton of the MR-TADF emitter on device degradation, blue TADF-OLED was fabricated with aid of a TTA host NPAN, the reduced triplet exciton concentration resulted in a long blue TADF-OLED lifetime with a LT95 of 420 hours at 1,000 cd/m2.
In addition to stable transport layer materials, the stability of the emitters has also been identified as a key factor influencing charge leakages. In 2019, Tanaka et al. investigated the impact of charge balance in the EML on the degradation mechanism of blue TADF-OLEDs[55]. Generally, TADF emitters with deeper LUMO energy levels compared to the host materials, and high doping concentrations, will affect the electron transport properties within the EML of TADF-OLEDs. Therefore, the degradation of the TADF emitters can disrupt the charge transport balance within the EML. By comparing the devices based on blue TADF emitters penta(9H-carbazol-9-yl)benzonitrile (5CzBN) with long triplet exciton lifetime and 2,4,6-tris(9H-carbaozle-9-yl)-3,5-bis(3,6-diphenylcarbazole-9-yl)benzonitrile (3Cz2DPhCzBN) with a shorter triplet exciton lifetime, they found that the decomposition of 5CzBN due to more severe TPA processes formed by deep electron traps, which caused the decreased electron transport capability. The carrier recombination zone was shifted towards the side of HBL, after which hole leaked into HBL, further leading to degradation of HBL. In contrast, 3Cz2DPhCzBN with better electron transport stability improved the charge transport balance, and thus the significantly extended the device stability. The device based on 3Cz2DPhCzBN exhibited a lifetime 25 times longer than the device based on 5CzBN, with a LT90 of 201 hours at 1,000 cd/m2. Additionally, the higher PLQY of 3Cz2DPhCzBN enabled a high EQEmax of over 20% for blue device. Therefore, maintaining long-term charge transport balance within the EML also suppresses degradation caused by charge leakage.
Preventing long-lived triplet excitons from diffusing out of the recombination zone and damaging functional materials can effectively improve the lifetime of blue TADF-OLEDs. In 2021, Chung et al. proposed a device structure that retains short-lived triplet excitons for emission while eliminating harmful long-lived triplet excitons[56]. By introducing a quenching layer (QL) outside the exciton recombination zone, long-lived triplet excitons that diffuse out of the recombination zone are effectively quenched. However, the short-lived triplet excitons for emission will not being quenched, because the quenching layer is located outside the exciton recombination zone. Accordingly, the blue TADF-OLED based on 5CzBN with a quenching layer achieved a high EQEmax of 22% and a LT60 of 114 hours at 500 cd/m2, doubling the lifetime compared to the device without the quenching layer.
Furthermore, suppressing the inevitable TTA and TPA processes that generate hot exciton can also improve the lifetime of blue TADF-OLEDs. In 2022, Lim et al. demonstrated that adding the polaron retarder fac-tris(5-(tert-butyl)-1,3-diphenyl-2,3-dihydro-1H-imidazo[4,5-b]pyrazine)iridium (Ir(cb)3) to an OLED based on the blue TADF emitter 5CzCN extended the lifetime of the blue TADF-OLED by controlling hot exciton relaxation and exciton-polaron dispersion[57]. The introduction of the polaron retarder optimized the distribution of excitons and polarons by capturing holes to improve charge balance. Additionally, the polaron retarder inhibited bond dissociation of host and emitter through exothermically energy transfer, and the contribution to lifetime extension was 41% from charge balance and 59% from hot exciton relaxation. As a result, the LT50 of the blue TADF OLED with the polaron retarder increased from 42 hours to 83 hours at 1,000 cd/m2 and the EQEmax increased from 15.8% to 20.1%.
Notably, one should also consider the quenching processes caused by singlet exciton accumulation since blue TADF-OLEDs rely on singlet exciton radiative transitions. In 2018, Liu et al. demonstrated that singlet-singlet annihilation (SSA) process is a significant degradation pathway in blue TADF-OLEDs[58]. They further derived from a diffusion-limited annihilation model that the decomposition rate of TADF emitters is proportional to the SSA process rate and inversely proportional to the square of the fluorescence rate (kF). Consequently, they designed and synthesized a series of blue TADF emitters with different kF, including 5-(4-(4,6-diphenyl-1,3,5-triazin-2-yl)phenyl)-12-phenyl-5,12-dihydroindolo[3,2-a]carbazole (DCz-TRZ), 3,3'',6,6''-tetra-tert-butyl-9'-(4-(4,6-diphenyl-1,3,5-triazin-2-yl)phenyl)-9'H-9,3':6',9''-tercarbazolet (tBu3Cz-TRZ), and 9-(4-(4,6-diphenyl-1,3,5-triazin-2-yl)phenyl)-9',9''-diphenyl-9H,9'H,9''H-3,3':6',3''-tercarbazole (TCz-TRZ). Among these materials, DCz-TRZ, which has a higher k F value, undergoes a faster radiative decay process, thus shortening the singlet exciton lifetime and reducing singlet exciton density. Consequently, the device based on DCz-TRZ achieved a high EQEmax of 13% and a LT50 of nearly 100 hours at 500 cd/m2.
In summary, charge transport balance is a prerequisite for high-performance blue TADF-OLEDs. Additionally, the rapid depletion of high-energy triplet excitons is an effective strategy for extending the lifetime of blue TADF-OLEDs. The degradation processes triggered by singlet excitons should also receive sufficient attention in certain device systems. The chemical structures of TADF emitters and transporting materials are shown in Figure 5 and Figure 6, respectively. The performances of devices based on blue fluorescent, phosphorescent and TADF emitters are shown in Table 1.
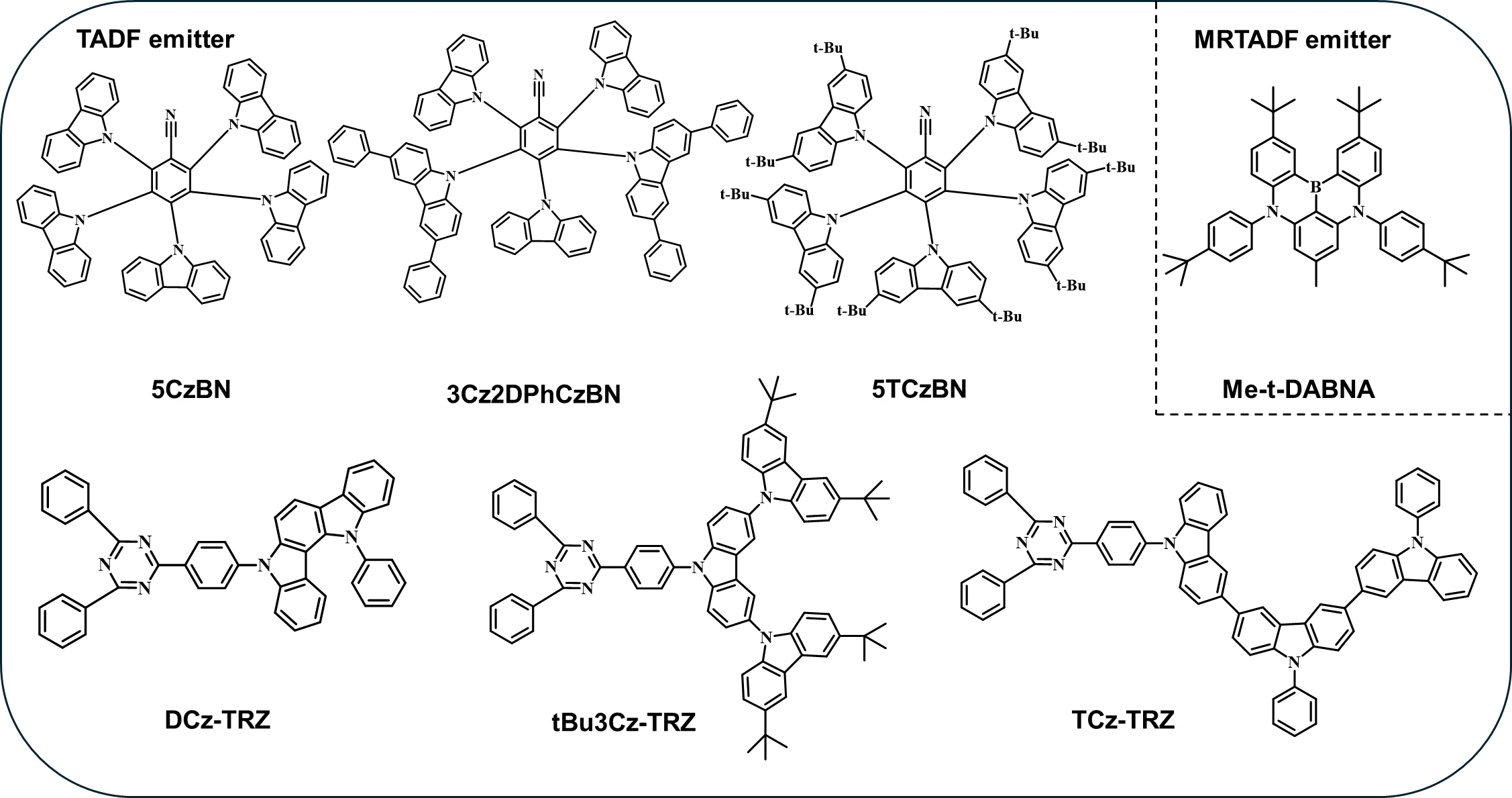
Figure 5. Chemical structures of TADF emitters. TADF: thermally activated delayed fluorescence.
Device structure | Von (V) | EQEmax (%) | Lifetime (h) | CIE (x,y) | Ref. |
Blue Fluorescent OLED | |||||
ITO/HAT-CN/BPBPA/PPMF-SF/ANDN: 3 wt% B-N-S-3/Na-An-Bi: Liq/Yb/Ag | 3.04 | 6.7 | LT95 = 136(1000 nit) | (0.128, 0.119) | [17] |
ITO/HAT-CN/Tris-PCz/NaNaP-A/SF3-TRZ/SF3-TRZ: 50 wt% Liq/Liq/Al | 2.8 | 6.2 | - | - | [26] |
ITO/HAT-CN/Tris-PCz/NaNaP-A: 1 wt% v-DABNA/SF3-TRZ/SF3-TRZ: 50 wt% Liq/Liq/Al | 2.8 | 8.0 | - | (0.12, 0.12) | [26] |
ITO/PEDOT: PSS/TCTA/CPPCN/TPBi/LiF/Al | - | 11.64 | - | (0.15, 0.17) | [34] |
Blue Phosphorescent OLED | |||||
ITO/HAT-CN/CPD/mCBP: 18 Vol%-14 Vol% Ir(dmp)3/mCBP: 11 Vol%-9 Vol% Ir(pmp)3: 3 Vol% mer-Ir(pmp)3/mCBP: 12 Vol%-8 Vol% Ir(dmp)3/mCBP: 8 Vol% Ir(dmp)3/mCBP/Alq3/Liq/Al | - | 9.6 | LT80 = 334(1000 nit) | (0.16, 0.30) | [40] |
ITO/DNTPD/BPBPA/PCZAC/mCBP: 10 wt%Ir(dbi)3/LG201/LiF/Al | - | 14.0 | LT70 = 180(500 nit) | (0.19, 0.44) | [42] |
ITO/DNTPD/BPBPA/mCBP: 10 wt% Ir(dbi)3/DBTP1: PBICT: 10 wt% Ir(ppy)2acac/ZADN/LiF/Al | - | 11.2 | LT70 = 47(1000 nit) | - | [43] |
ITO/HAT-CN/mCBP: 18 Vol%-8 Vol% Ir(dmp)3/mCBP/Alq3/Liq/Al | - | 9.5 | LT80 = 213(1000 nit) | (0.16, 0.31) | [44] |
ITO/HAT-CN/mCBP: 18 Vol%-8 Vol% Ir(dmp)3/mCBP/Alq3/Alq3: 2 Vol% Li/HAT-CN/mCBP: 18 Vol%-8 Vol% Ir(dmp)3/mCBP/Alq3/Liq/Al | - | 18 | LT80 = 616(1000 nit) | (0.15, 0.29) | [44] |
ITO/HAT-CN/NPD/Tris-PC/mCBP: 20 wt% PtNON/mCBP: 10 wt% PtNON/mCBP: 6 wt% PtNON/mCBT/BPyTP/LiF/Al | 3.41 | 17.4 | LT70 = 1337(1000 nit) | (0.19, 0.41) | [45] |
ITO/BPBPA: 30 wt% HATCN/BPBPA/PCzAC/mCBP: 5 wt% iPrImIr/mCBP: 5 wt% CNImIr/DBFTrz/ZADN/LiF/Al | - | 16.1 | LT95 = 13.8(1000 nit) | (0.15, 0.24) | [46] |
ITO/DNTPD/BPBPA/PCZAC: 10 wt% Ir(fim)3/mCBP: 10 wt% Ir(dbi)3/ZADN/LiF/Al | - | 13.0 | LT70 = 118(1000 nit) | - | [48] |
Blue TADF-OLED | |||||
ITO/PPBI/NPD/4DBFHPB/mCBP: 30 wt% 5TCzBN/DBT-TRZ/DBP: 20 wt% Liq/Libpp/Al | 2.74 | 21.5 | LT50 = 1700(500 nit) | - | [53] |
ITO/HAT-CN/DBF-SFX/mCBP: 3 wt% M-t-DABNA/DPEPO/TmPyPb/LiQ/Al | 3.8 | 33.2 | LT90 = 7.2(100 nit) | (0.13, 0.10) | [54] |
ITO/HATCN/DBF-SFX/EBM/NPAN: 3 wt% M-t-DABNA/HBM/AIT-BIZ: Liq (1:2)/Yb/Ag | - | - | LT95 = 420(540 nit) | (0.13, 0.10) | [54] |
ITO/HAT-CN/Tris-PCz/mCBP/mCBP: 20 wt% 3Cz2DPhCzBN/SF3-TRZ/SF3-TRZ: 30 wt% Liq/Liq/Al | - | 20.0 | LT90 = 201(1000 nit) | - | [55] |
ITO/DNTPD/BCFN/mCBP/mCBP: CNDBF2CNCz: TPBe (50:50:1)/mCBP: CNDBF2CNCz: 5CZCN (50:50:20)/DBFTrz/ZADN/LiF/Al | - | 22 | LT60 = 114(500 nit) | (0.17, 0.36) | [56] |
ITO/DNTPD/BCFA/mCBP/mCBP: CN-mCBPCN: Ir(cb)3: 5CzCN (50:50:3:10)/DBFTrz/ZADN/LiF/Al | 3.0 | 20.1 | LT50 = 83(1000 nit) | (0.16, 0.30) | [57] |
ITO/HAT-CN/NPB/TCTA/mCBP/DPEPO: 30 wt% DCz-TRZ/TPBi/Liq/Al | 3.3 | 15.5 | LT50 = 100(500 nit) | (0.16, 0.38) | [58] |
TADF: thermally activated delayed fluorescent; OLEDs: organic light-emitting diodes; EQE: external quantum efficiency; CIE: Commission Internationale de l'Eclairage.
3.4 Host engineering for high-performance and long-lifetime blue OLEDs
The properties of the host materials, such as charge transport, HOMO and LUMO levels, and singlet and triplet energy levels, play a crucial role in regulating charge and exciton behaviors. Consequently, a series of hosts such as traditional fluorescent type host, TTA/hot exciton type host, TADF-assistant host, phosphorescent-assistant host, and exciplex/electroplex hosts were categorized and discussed.
3.4.1 Traditional fluorescent type host
Traditional fluorescent type hosts with high triplet energy can be classified into n-type, p-type and bipolar hosts based on their charge transport properties. The n-type host exhibits strong electron transport properties, while the p-type host is characterized by strong hole transport properties. Bipolar hosts are capable of efficiently transporting both electrons and holes[59]. Additionally, mixing n-type and p-type hosts in an appropriate proportion can achieve balanced charge transport and a uniformed emission zone. As mentioned above, the difference between the HOMO and LUMO levels of host and emitter has significantly influence in charge transport[60]. Therefore, considering the charge transport properties of the selected blue emitters, a traditional fluorescent host material with complementary charge transporting characteristic is beneficial for constructing high-performance and long-lifetime blue OLEDs. The chemical structures of traditional fluorescent type hosts are shown in Figure 7. The performances of devices based on traditional fluorescent hosts are shown in Table 2.
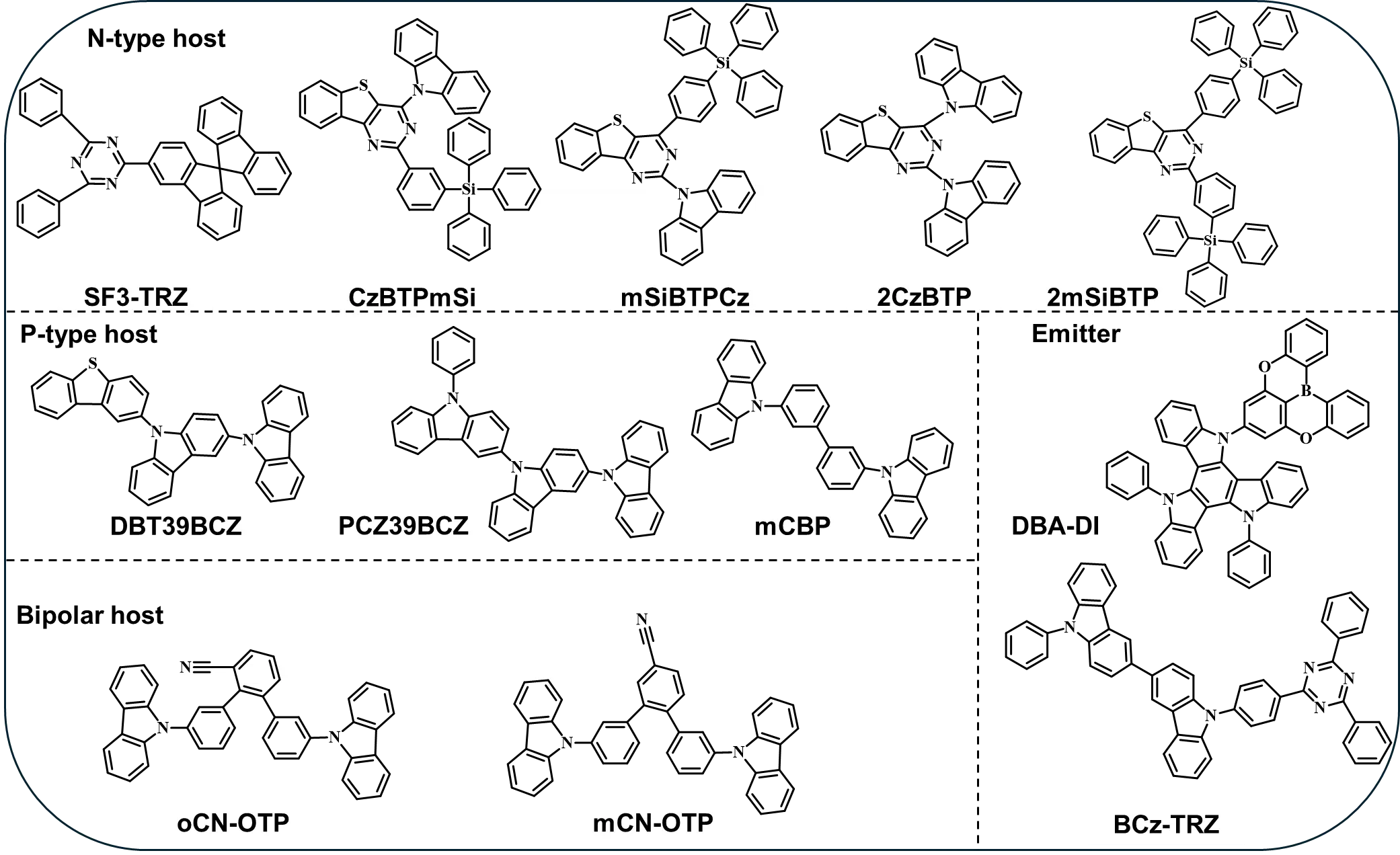
Figure 7. Chemical structures of n-type hosts, p-type hosts, bipolar hosts and emitters.
Device structure | Von (V) | EQEmax (%) | Lifetime (h) | CIE (x,y) | Ref. |
ITO/HAT-CN/α-NPD/Tris-PCz/mCBP/SF3-TRZ: 30 wt% BCz-TRZ/SF3-TRZ/Bebq2/LiF/Al | 2.62 | 8.8 | LT50 = 454(1,000 nit) | (0.20, 0.36) | [61] |
ITO/HAT-CN/BPBPA/PCZAc/DBT39PCZ: 5 wt% Ir(dbi)3/DBFTrz/NAPIm/LiF/Al | - | 20 | LT60 = 50(1,000 nit) | - | [62] |
ITO/BPBPA: 30 wt% HATCN/BPBPA/mCBP/mCBP: CZBTPmSi: CNImIr (50:50:15)/DBFTrz/ZADN/LiF/Al | 2.3 | 23.9 | LT50 = 4364(100 nit) | (0.15, 0.27) | [63] |
ITO/HATCN/NPB/PCZAC/oCN-OTP: 30 wt% DBA-DI/DDBFT/BPPB/LiF/Al | 2.9 | 29.5 | LT90 = 28.8(1,000 nit) | (0.16, 0.38) | [64] |
EL: electroluminescence; OLEDs: organic light-emitting diodes; EQE: external quantum efficiency; CIE: Commission Internationale de l'Eclairage.
In 2017, Cui et al. demonstrated that n-type hosts are more suitable than p-type hosts for high-performance and long-lifetime blue TADF-OLEDs[61]. N-type hosts possess deeper HOMO levels and excellent electron transport capabilities, When hole transporting dominant bipolar TADF emitters are combined with electron transporting dominant n-type hosts, they help ensure balanced charge transportation. Additionally, excitons are generally generated on the blue emitters through charge trapping to avoid high-energy excitons formed on the host and consequently suppress the decomposition of unstable chemical bonds. Furthermore, balanced charge transport within the EML contributes to a broadened recombination zone, which reduces the density of triplet excitons and excess polarons. As a result, the blue OLED incorporating 9-(4-(4,6-diphenyl-1,3,5-triazin-2-yl)phenyl)-9'-phenyl-9H,9'H-3,3'-bicarbazole (BCz-TRZ) as the TADF emitter and SF3-TRZ as the n-type host achieved an EQEmax of 8.8% and a LT50 of 454 hours at 1,000 cd/m2.
Notably, it is essential to ensure that the unipolar transporting host remains stable in anionic or cationic state to achieve high-efficiency and long-lifetime blue OLEDs. In 2017, Byeon et al. developed two p-type hosts, DBT39BCZ and PCZ39BCZ for developing high-performance blue phosphorescent OLEDs based on the emitter Ir(dbi)3. Both hosts possess high triplet energy and exhibit good stability against negative polarons[62]. In comparison, the device based on DBT39BCZ exhibited better charge transport balance and a broader emission zone, and thus an EQEmax of over 20% with low efficiency roll-off (an EQE of over 17% at 1,000 cd/m2) were achieved. Additionally, the weak C-N bond of DBT39BCZ has a higher BDE in the negative polaron state, and thus a longer device lifetime of LT60 of over 50 hours at 1,000 cd/m2 was achieved.
Additionally, the impact of traditional fluorescent hosts on the performance of blue OLEDs is primarily related to providing suitable charge transport properties. A mixed-host strategy is often employed to improve carrier balance and disperse excitons within the EML. In 2020, Kim et al. developed four positive polaron stabilizing n-type hosts 4-(9H-carbazol-9-yl)-2-(3-(triphenylsilyl)phenyl)benzo[4,5]thieno[3,2-d]pyrimidine (CzBTPmSi), 2-(9H-carbazol-9-yl)-4-(3-(triphenylsilyl)phenyl)benzo[4,5]thieno[3,2-d]pyrimidine (mSiBTPCz), 2,4-di(9H-carbazol-9-yl)benzo[4,5]thieno[3,2-d]pyrimidine (2CzBTP), and 2,4-bis(3-(triphenylsilyl)phenyl)benzo-[4,5]thieno[3,2-d]pyrimidine (2mSiBTP), and furtherly incorporating with mCBP to create a mixed host for high-performance blue phosphorescent OLEDs based on the phosphorescent emitter CNImIr (Figure 8a)[63]. Benefit from the balanced charge transport and excellent stability, all these devices achieved a low efficiency roll-off and a long device lifetime (LT50 exceeding 1,000 hours at 100 cd/m2). Especially for CzBTPmSi-based device, a LT50 of 4,364 hours at 100 cd/m2 was realized (Figure 8b). Additionally, owing to the high triplet energies, the devices based on CzBTPmSi, 2CzBTP, and 2mSiBTP achieved EQEmax exceeding 20% (Figure 8c). While the device based on mSiBTPCz host exhibited a reduced EQEmax of 16.1%, which is attributed to the lower triplet energy causing reverse energy transfer from the phosphorescent emitter to the host.
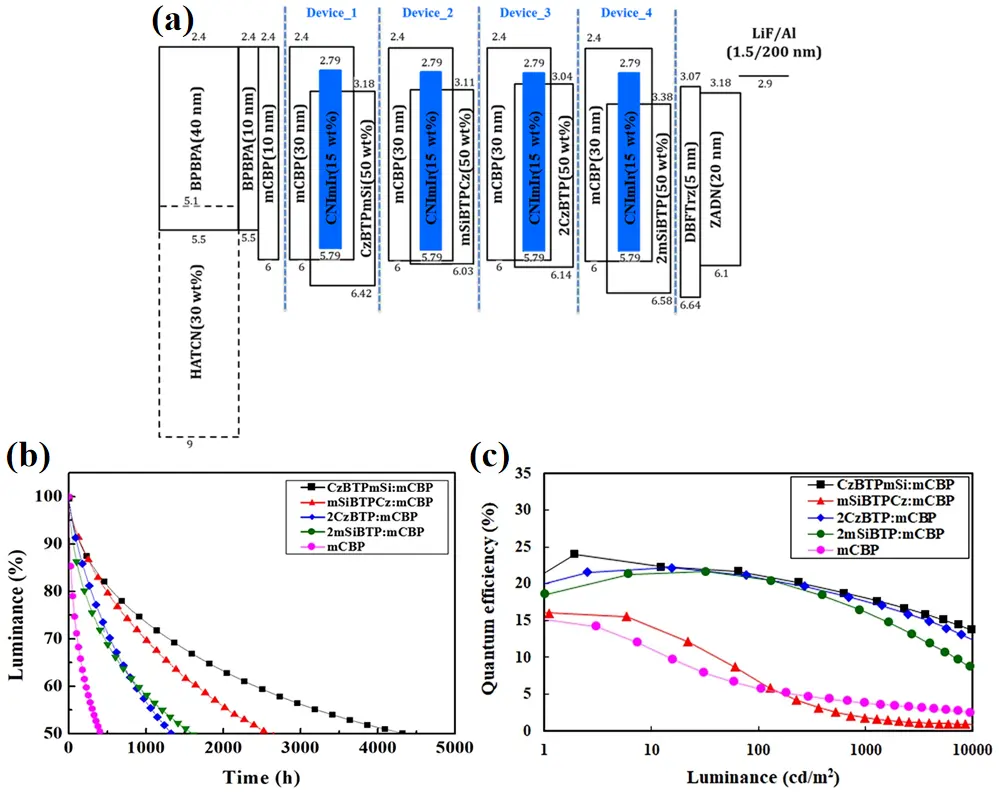
Figure 8. (a) Device structure and energy level diagram of the device; (b) lifetime data at 100 cd/m2; (c) EQE-luminance curves. Republished with permission from[63]. EQE: external quantum efficiency.
A high triplet energy, stable bipolar host can also replace mixed hosts to achieve high-performance blue OLEDs. In 2021, Lee et al. developed two bipolar transport hosts, ortho-substituted 3,3''-di(9H-carbazol-9-yl)-[1,1':2',1''-terphenyl]-3'-carbonitrile (oCN-OTP) and meta-substituted 3,3''-di(9H-carbazol-9-yl)-[1,1':2',1''-terphenyl]-4'-carbonitrile (mCN-OTP) for high-performance blue TADF-OLEDs based on the emitter 5-(5,9-dioxa-13b-boranaphtho[3,2,1-de]anthracen-7-yl)-10,15-diphenyl-10,15-dihydro-5H-diindolo[3,2-a:30,20-c]carbazole (DBA-DI)[64]. Compared to mCN-OTP, oCN-OTP host exhibits more balanced charge transport and broadened recombination zone. As a result, the blue TADF-OLED based on oCN-OTP achieved a EQEmax of 29.5% with low efficiency roll-off (with an EQE of 28.7% at 1,000 cd/m2) and a LT90 of 28.8 hours at 1,000 cd/m2, which was obviously superior to the device based on mCN-OTP host (with an EQEmax of 21.9% and a LT90 of 9 hours at 1,000 cd/m2).
3.4.2 TTA/hot exciton type host
Compared to traditional fluorescent type host, TTA materials offer a higher EUE as host materials. Specifically, the triplet excitons on TTA hosts can be harvested through the TTA process, which generates additional singlet excitons. The energy of these singlet excitons can then be transferred to the blue emitters via Förster resonance energy transfer (FRET) process. Consequently, TTA hosts are typically employed in high-performance, long-lifetime blue OLEDs due to their higher EUE and excellent stability[65-67]. In addition, hot exciton materials with 100% EUE are also suitable for using as hosts in blue OLEDs. High-energy triplet excitons on hot exciton hosts are rapidly converted into singlet excitons through the hRISC channel, after which the singlet exciton on the hot exciton host is transferred to the blue emitter via the FRET process[33,68,69]. The rapid hRISC process can suppress the Dexter energy transfer (DET) interaction to reduce the accumulation of triplet excitons on the emitter. Therefore, the impact of TTA hosts and hot exciton hosts on the performance of blue OLEDs is primarily reflected in exciton utilization and stability. The chemical structures of TTA/hot exciton hosts and functional materials are shown in Figure 9. The performances of devices based on TTA/hot exciton hosts are shown in Table 3.
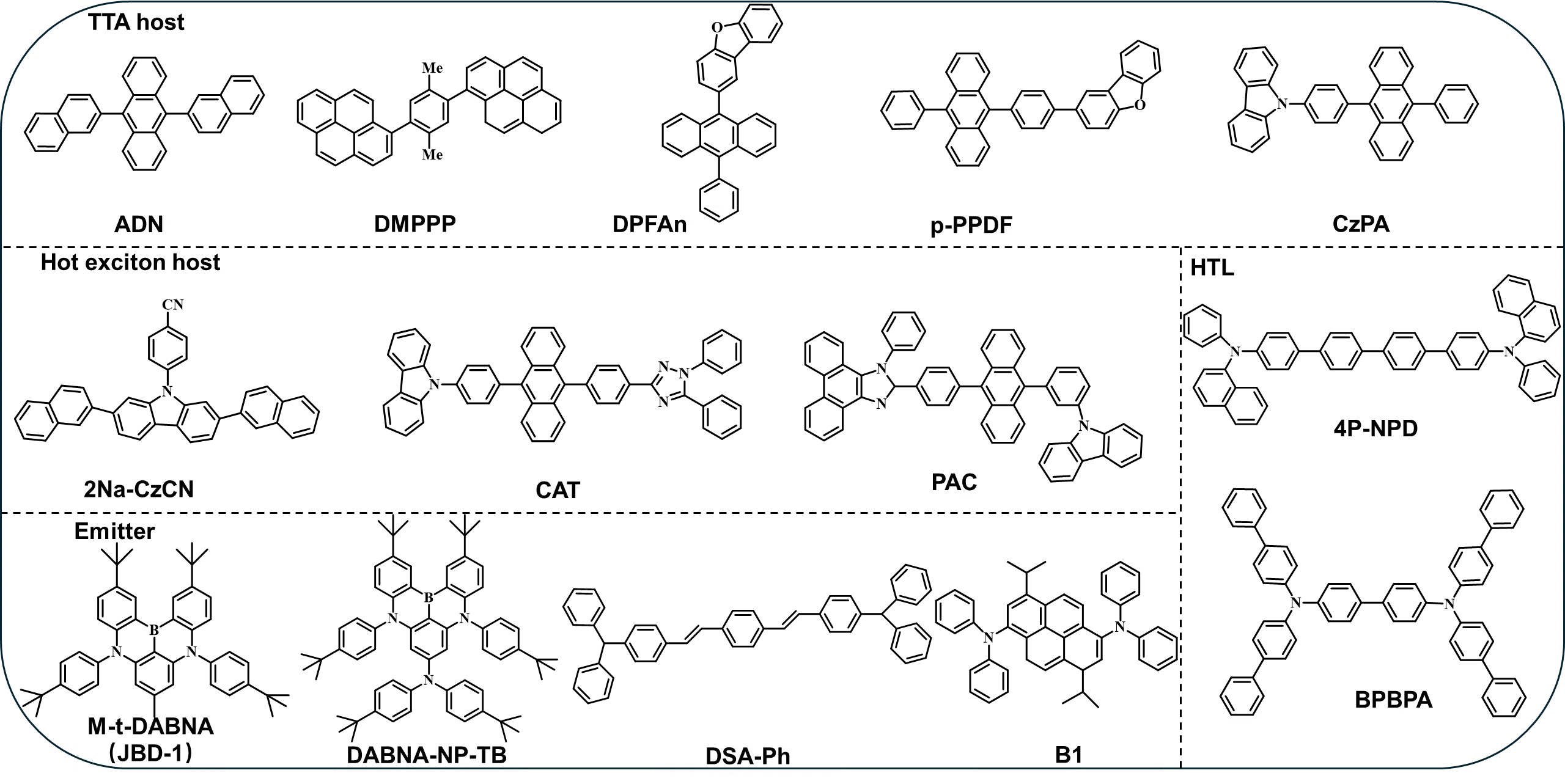
Figure 9. The chemical structures of TTA/hot exciton hosts and functional materials. TTA: triplet-triplet annihilation.
Device structure | Von (V) | EQEmax (%) | Lifetime (h) | CIE (x,y) | Ref. |
ITO/TAPC/TCTA/MADN: 1 wt% MBD106/MADN: 3 wt% MBD106/ADP3Py/B3PyMPM/Cs2CO3/Al | 2.7 | 10.2 | - | (0.134, 0.131) | [70] |
ITO/TAPC/TCTA/MADN: 1 wt% M-tDABNA/MADN: 3 wt% M-tDABNA/ADP3Py/B3PyMPM/Cs2CO3/Al | 2.7 | 8.6 | - | (0.137, 0.076) | [70] |
ITO/MoO3/BATCP/mBCpBC/MADN: 1 wt% MBD106/MADN: 3 wt%MBD106/ADP3Py/DPPyA: Liq (1:1)/Liq/Al | - | 7.29 | LT50 = 750(400 nit) | (0.134, 0.155) | [70] |
ITO/HAT-CN/TAPC/TCTA/CzPA: 2 wt% DSA-ph/2Na-CzCN/BmPyPb/LiF/Al | 2.6 | 15.2 | LT50 = 40(1,000 nit) | (0.14, 0.24) | [71] |
ITO/HAT-CN/Tris-PCz/1,2-AND: 5 wt% HDT-1: 1 wt% v-DABNA/1,2-AND: 1 wt% v-DABNA/SF3-TRZ/SF3-TRZ: 50 wt% Liq/Liq/Al | 2.8 | 5 | LT90 = 120(1,000 nit) | (0.12, 0.13) | [72] |
ITO/HAT-CN/PCBBiF/DMPPP: 7 wt% M-t-DABNA/DPFAn: 5 wt% M-t-DABNA/BPPB/LiF/Al | 3.3 | 10.8 | - | (0.13, 0.09) | [75] |
ITO/HAT-CN/PCBBiF/P-PPDF: 7 wt% DABNA-NP-TB/DPFAn: 5 wt% DABNA-NP-TB/BPPB/LiF/Al | 3.3 | 10.4 | LT95 = 79(1,000 nit) | (0.13, 0.07) | [75] |
ITO/MoO3/mCBP/CAT: 2 wt% B1/NBphen: Liq (1:1)/LiF/Al | 2.8 | 9.13 | LT50 = 320(540 nit) | (0.138, 0.157) | [76] |
ITO/HATCN/NPB/BCzPH/PAC: 1 wt%JBD-1/NBPhen/LiF/Al | 2.5 | 4.7 | LT50 = 458(1,000 nit) | (0.13, 0.11) | [77] |
EL: electroluminescence; OLEDs: organic light-emitting diodes; TTA: triplet-triplet annihilation; EQE: external quantum efficiency; CIE: Commission Internationale de l'Eclairage.
A narrow recombination zone can accumulate triplet excitons, thereby enhancing the TTA process. In 2022, Lim et al. broke the efficiency limits of anthracene-derived blue TTA-OLED by utilizing a device structure with an efficiency-enhancement layer (EEL)[70]. The optimized device structure with an EEL was shown in Figure 10a. By regulating charge transport, carrier recombination occurs within a narrow EEL (ADP3Py), where a significant accumulation of triplet excitons rapidly facilitates the TTA process. Additionally, the singlet excitons generated on the EEL are transferred to the blue emitter in the EML through the FRET process. The devices using the traditional fluorescent emitter MBD106 and the MR-TADF emitter M-tDABNA as terminal emitters achieved EQEmax of 10.2% and 8.6%, respectively. Transient EL decay emission results indicated that the total radiative exciton ratio in blue TTA-OLEDs based on the MBD106 emitter reached 48%, breaking the theoretical limit (40%). As shown in Figure 10b,c, the efficient TTA process and extra RISC process from T5 to S1 on the EEL are key to surpassing the theoretical efficiency limit. Unfortunately, the poor stability of some functional layers in the device resulted in a short operational lifetime. When a stable device structure was employed, the LT50 at 400 cd/m2 reached 750 hours, but the contribution of TTA to delayed emission was only 22%.
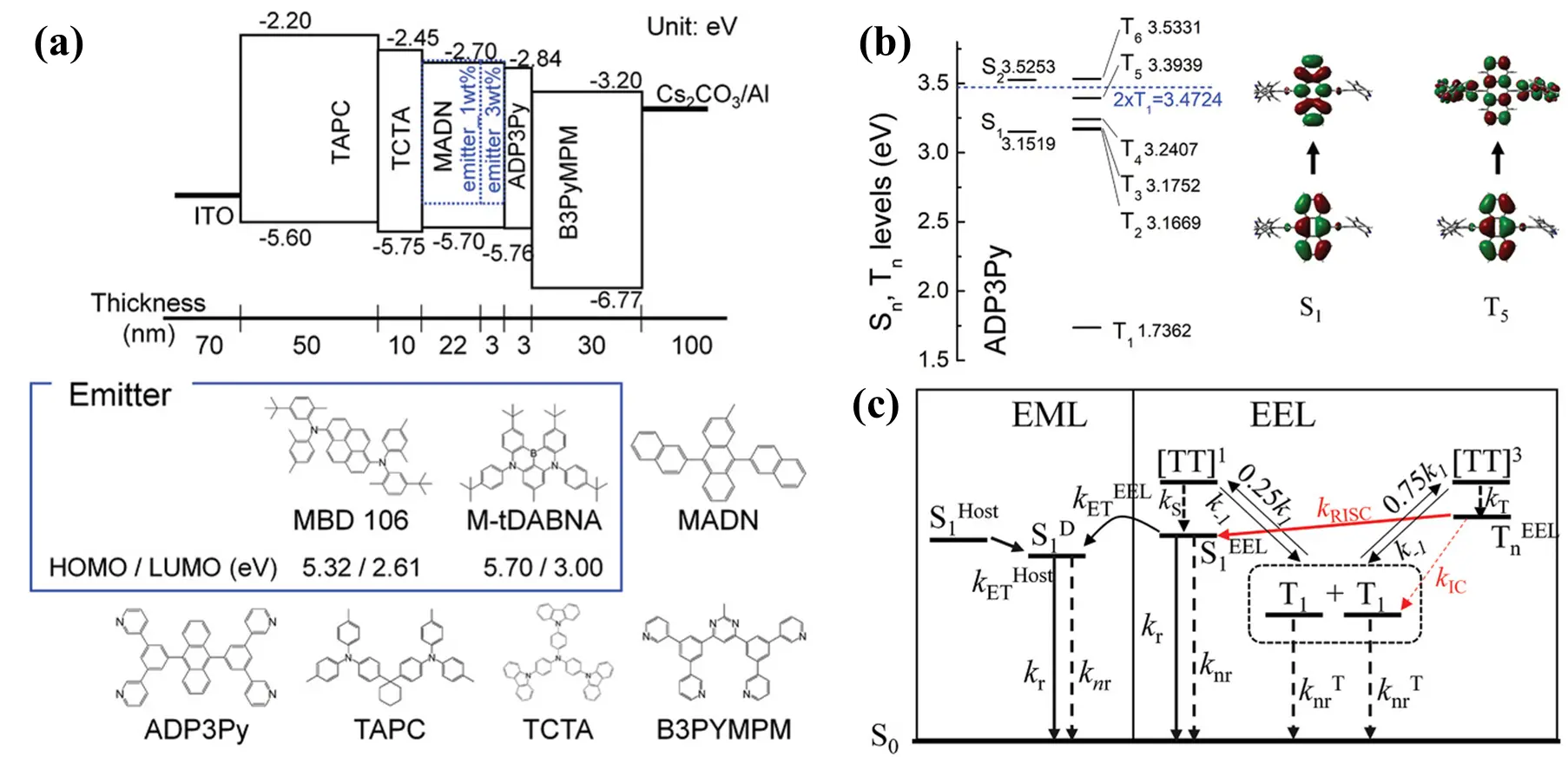
Figure 10. (a) Device structure with energy levels and thicknesses and molecular structures of materials used in the device; (b) simulated singlet and triplet levels and NTOs corresponding to the S1 and T5 state of ADP3Py using B3LYP functional and 6-31G(d) basis set; (c) EQE against current density of the devices for two different emitters of MBD and M-tDABNA doped in MADN host with the doping concentration of 3 wt%. Republished with permission from[70]. EQE: external quantum efficiency.
Considering that hot exciton materials inherently possess hRISC channels and have a theoretical EUE that exceeds that of TTA materials. In 2024, Xie et al. inserted a "hot exciton" EEL between the EML and ETL in blue TTA-OLED[71]. The EML is composed of blue fluorescent material DSA-Ph (2 wt%) and TTA host CzPA, while the non-doped hot exciton material 2Na-CzCN forms the EEL. The triplet excitons on Tn of 2Na-CzCN are transferred to its S1 via the hRISC process. Subsequently, the singlet excitons in 2Na-CzCN are stepwise transferred via Förster Energy Transfer (FET) process from CzPA to the DSA-Ph. In addition, the triplet excitons on Tn of 2Na-CzCN can be converted to the T1 state through IC process. The lower-energy triplet excitons in CzPA can harvest the lost IC excitons for the TTA process. As a result, the excitons generated in the EEL are fully harvested. Therefore, a high EQEmax of 15.2% (4,000 cd/m2), a maximum luminance of 80,706 cd/m2, and a LT50 of 40 h at 1,000 cd/m2 were achieved, which showed 5.7-fold increase in device lifetime compared to devices without the EEL.
Moreover, Nguyen et al. demonstrated that an efficient TTA process can also be achieved in a broad recombination zone[72]. They introduced HDT-1, a D-A type TADF material, as an assistant dopant in the EML of blue TTA-OLEDs. Excitons are directly formed on the HDT-1, and triplet excitons are quickly quenched by TTA host 1,2-ADN. This creates a localized region around each HDT-1 molecule with a high concentration of triplet excitons, facilitating an efficient TTA process and bringing the TTA efficiency close to its theoretical maximum. In addition, by introducing HDT-1 with bipolar transport capabilities, the high concentration of triplet excitons can diffuse from the narrow interface region to a larger portion of the EML. Consequently, the blue TTA-OLED based on blue multi-resonant thermally activated delayed fluorescent (MRTADF) emitter v-DABNA achieves a LT90 of over 120 hours at 1,000 cd/m2, which is five times longer than devices without HDT-1. However, the blue device achieved an EQEmax of only 5%, which is a 30% decrease compared to the device without HDT-1. It appears that there is always a trade-off between high efficiency and long lifetime in blue OLEDs based on TTA hosts.
In 2019, Kang et al. investigated the trade-off between efficiency and lifetime in blue OLED with TTA host ADN[73]. Initially, devices based on two HTMs, N4,N4,N4',N4'-tetra([1,1'-biphenyl]-4-yl)-[1,1'-biphenyl]-4,4'-diamine (BPBPA) with weaker hole transport capability and N,N'-di-1-naphthalenyl-N,N'-diphenyl-[1,1':4',1'':4'',1'''-quaterphenyl]-4,4''' diamine (4P-NPD) with stronger hole transport capability, were fabricated to study operational stability. The device using BPBPA as the HTL exhibits relatively unbalanced charge transport, leading to significant electron leakage, although the contribution of TTA to delayed fluorescence is higher. As a result, this device shows high efficiency but reduced operational lifetime. In contrast, the device utilizing 4P-NPD as the HTL achieves more balanced charge transport, but the reduced triplet exciton density diminishes the TTA process. In summary, the trade-off between efficiency and lifetime in blue OLEDs based on TTA hosts can be served as the conflict between a narrow TTA region and a broad exciton recombination region. By managing charges and excitons to separate the TTA region from the exciton recombination region maybe resolve this conflict.
In 2022, Tasaki et al. introduced the concept of a bilayer EML in blue TTA-OLED[74]. Two blue host materials with strong electron transport capabilities make the majority of excitons are generated in EML1. As shown in Figure 11a, triplet excitons in EML1 are transferred to EML2 through DET process, where the TTA process occurs. Since two hosts with close S1 energy levels, singlet excitons can undergo radiative decay in both EML1 and EML2. Consequently, the EML is divided into a charge recombination zone (EML1) and a TTA zone (EML2). The suppression of localized degradation and the dispersion of the emission region contribute to the extended device lifetime. Therefore, blue TTA-OLED with a bilayer EML achieved a high EQEmax of 12% and a LT95 of 450 hours at a constant current density of 50 mA/cm2 (Figure 11b,c).
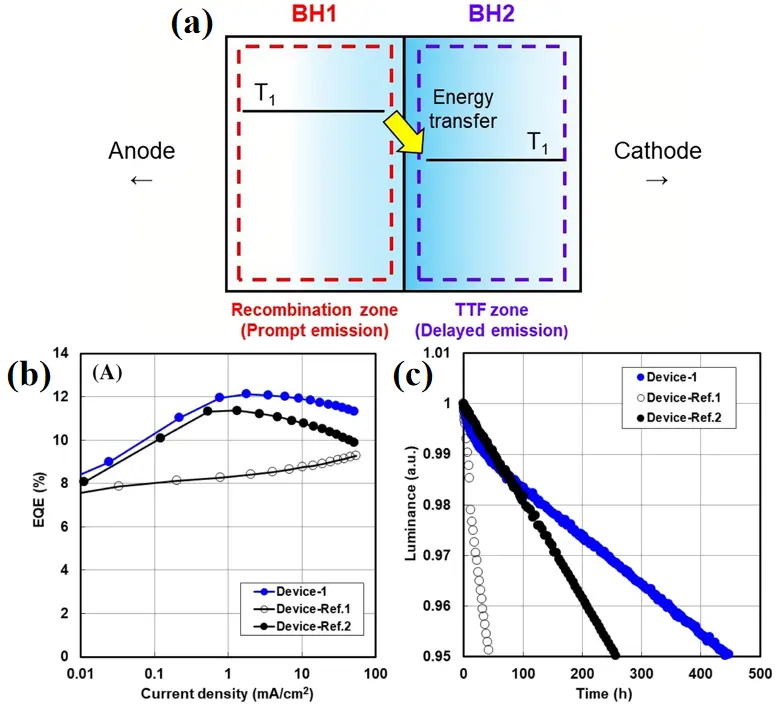
Figure 11. (a) Concept of bilayer emitting layer; (b) EQE against current density of the devices; (c) lifetime measurement of the devices at a current density of 50 mA/cm2. Republished with permission from[74]. EQE: external quantum efficiency; TTF: three tetrathiafulvalene.
Furthermore, in 2023, Lee et al. investigated a blue OLED with a d-EML structure based on TTA hosts and a pure blue MR-TADF emitter[75]. In EML1, the TTA host 1,10-(2,5-dimethyl-1,4-phenylene)dipyrene (DMPPP) with a higher triplet energy level was used, while in EML2, the TTA host 2-(10-phenylanthracen-9-yl)dibenzo[b,d]furan (DPFAn) was employed, with both EMLs doped with the MR-TADF emitter M-t-DABNA. Using d-EML strategy, the TTA proportion reached 32.4%, close to the theoretical limit of 37.5%, achieving a high EQEmax of 10.8% and a pure blue emission with CIE coordinates of (0.13, 0.09). Additionally, to enhance the lifetime of blue MR-TADF-OLEDs, the host in EML1 was replaced with 2-(4-(10-phenylanthracen-9-yl)phenyl)dibenzo[b,d]furan (p-PDDF) that has a deeper LUMO level, and the emitter was replaced with the deeper blue MR-TADF emitter 2,12-di-tert-butyl-N,N,5,9-tetrakis(4-(tert-butyl)phenyl)-5,9-dihydro-5,9-diaza-13b-boranaphtho[3,2,1-de]anthracen-7-amine (DABNA-NP-TB). Compared to the single EML device, the d-EML structure significantly improved both the efficiency and the lifetime of the blue OLED, an EQEmax of 10.4% and LT95 of 79 hours at 1,000 cd/m2 was achieved, with a pure blue color coordinate of (0.13, 0.07) and narrow FWHM of 26 nm.
For blue OLEDs based on hot exciton hosts, the inability to harvest T1 excitons formed through the IC process, along with directly formed T1 excitons, leads to exciton loss. An important strategy to address this issue is to increase the pathways for utilizing T1 excitons. In 2022, Li et al. developed a hot exciton material CAT, employing an "exciton recovery" strategy to harvest these lost triplet excitons[76]. CAT is capable of harvesting high-lying triplet excitons through the hRISC channel. In addition, the directly generated triplet excitons and triplet excitons from the IC process, can be upconverted to singlet excitons through the TTA process. Based on this dual channels triplet exciton utilization strategy, the device achieved a high EQEmax of 10.39%, with a deep blue CIE color coordinate of (0.15, 0.087). Moreover, using CAT as hot exciton host for the blue fluorescent emitter B1, the device achieved an EQEmax of 9.13% and an LT50 of 320 hours at 540 cd/m2.
Similarly, in 2024, Yang et al. utilized the hot exciton material 2-(4-(10-(3-(9H-carbazol-9-yl)phenyl)anthracen-9-yl)phenyl)-1-phenyl-1H-phenanthro[9,10-d]imidazole (PAC), which has dual channels triplet exciton harvesting ability, as the host for the narrowband MR-TADF material methyl-2,12-di-tert-butyl-5,9-bis(4-(tert-butyl)phenyl)-5,9-dihydro-5,9-diaza-13b-boranaphtho[3,2,1-de]anthracene (JBD-1), demonstrating a stable narrow-band emission in blue fluorescent OLED[77]. The PAC host possesses a higher S1 and a lower T1 compared to JBD-1, allowing the rapid quenching of the long-lived triplet excitons on JBD-1 to avoid the detrimental annihilation processes. The PAC host enhances triplet exciton utilization by converting triplet excitons from the T2 to the S1 level via the RISC channel. Additionally, triplet excitons directly generated on PAC and those at the T1 produced through IC channel are harvested through the TTA process. Consequently, the device achieved an EQEmax of 7.6% and a LT50 of 458 hours at 1,000 cd/m2.
3.4.3 TADF assistant host
For blue OLEDs, host materials with high triplet energy are essential to prevent reverse energy transfer and to ensure efficient exciton utilization. However, the generation of high-energy singlet and triplet excitons on the host significantly impacts the stability of the EML materials. The strategy of introducing an assistant host with triplet energy between that of the host and the emitter is often used to address this issue. A high doping concentration enables the assistant host to become the primary site for exciton formation, thereby reducing the formation of higher-energy excitons on the host. Furthermore, effective energy transfer from the assistant host to the emitter ensures efficient exciton utilization. TADF materials, with high triplet exciton utilization efficiency, are often employed as assistant hosts. Notably, the RISC process of the TADF assistant hosts greatly influences the singlet-to-triplet exciton ratio on the emitter, which in turn affects both EUE and exciton quenching processes[78]. Therefore, TADF assistant hosts with a fast RISC process are favorable for achieving high-performance and long-lifetime blue OLEDs. The chemical structures of TADF assistant hosts and emitters are shown in Figure 12. The performances of devices based on TADF assistant hosts are shown in Table 4.
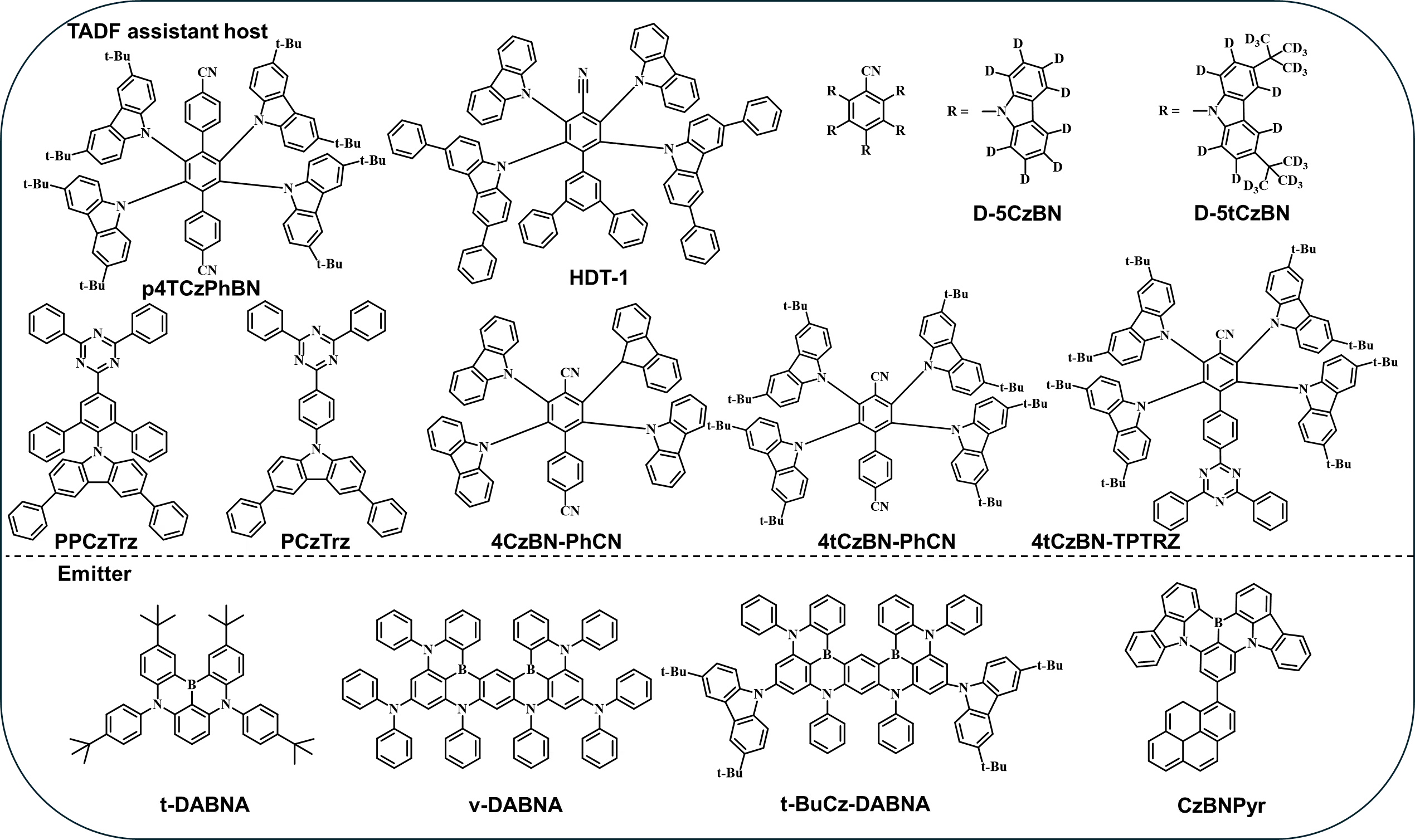
Figure 12. The chemical structures of TADF assistant hosts and emitters. TADF: thermally activated delayed fluorescence.
Device structure | Von (V) | EQEmax (%) | Lifetime (h) | CIE (x,y) | Ref. |
ITO/HATCN/NPB/TCTA/mCPCz: 40 wt% p4TCzPhBN: 2 wt% t-DABNA/CzPhPy/DPPyA: Liq (1:1)/LiF/Al | - | 32.5 | LT80 = 60(1000 nit) | (0.13, 0.12) | [79] |
ITO/HATCN/TAPC/Tris-PCz/mCBP/mCBP: 20 wt% HDT-1: 0.5 wt% v-DABNA/SF3-TRZ/SF3-TRZ: Liq/Liq/Al/HATCN/TAPC/Tris-Pcz/mCBP/mCBP: 20 wt% HDT-1: 0.5 wt% v-DABNA/SF3-TRZ/SF3TRZ: Liq/Liq/Al | 6.5 | 41.0 | LT95 = 18(1000 nit) | (0.13, 0.16) | [80] |
ITO/DNTPD/BPBPA/mCBP/oCBP: CNmCBPCN: PCz-Trz: v-DABNA (50:50:10:1)/DBFTrz/ZADN/LiF/Al | - | 33.5 | LT50 = 113(1000 nit) | (0.12, 0.18) | [83] |
ITO/DNTPD/BPBPA/mCBP/oCBP: CNmCBPCN: PPCz-Trz: v-DABNA (50:50:10:1)/DBFTrz/ZADN/LiF/Al | - | 33.0 | LT50 = 151(1000 nit) | (0.13, 0.20) | [83] |
ITO/HATCN/NPD/TCTA/mCBP/mCBP: 20 wt% HDT-1: 0.3 wt% CzBNPyr/SF3-TRZ/SF3-TRZ: 50 wt% Liq/Liq/Al | 3.2 | 19.4 | LT90 = 69.2(1000 nit) | (0.133, 0.318) | [84] |
EL: electroluminescence; OLEDs: organic light-emitting diodes; TADF: thermally activated delayed fluorescent; EQE: external quantum efficiency; CIE: Commission Internationale de l'Eclairage.
The blue TADF assistant hosts must exhibit a fast RISC process to efficiently and rapidly harvest triplet excitons rapidly. In 2020, Zhang et al. reported a deep blue TADF material, p4TCzPhBN (peaked at 456 nm), which exhibits a high RISC rate (kRISC) of 23.63 × 105 s-1[79]. The efficient RISC process enabled the blue TADF-OLED based on p4TCzPhBN to achieve a high EQE of 22.8% and a LT80 of 40.4 hours at 500 cd/m2. Further, p4TCzPhBN was employed as an assistant host for MR-TADF emitter 12-di-tert-butyl-5,9-bis(4-(tert-butyl)phenyl)-5,9-dihydro-5,9-diaza-13b-boranaphtho[3,2,1-de]anthracene (t-DABNA) which possesses a high radiative transition rate (kr) to fabricate deep blue narrowband OLEDs. The efficient RISC process of TADF assistant host enables the efficient conversion of triplet excitons and were subsequently consumed for rapid emission, and thus the reduced triplet exciton concentration and the improved exciton utilization. As a result, the device achieved a high EQEmax of 32.5% (The EQE is of 23.2% at 1,000 cd/m2), a LT80 of over 60 hours at 1,000 cd/m2, with a FWHM of 29 nm and a CIEy of 0.12.
Compared to deep blue TADF assistant hosts, stable sky-blue TADF assistant hosts that feature fast RISC process offer greater advantages in constructing a stable EML. Additionally, MRTADF emitters with small Stokes shift can reduce the necessity for assistant hosts with high exciton energy. Thus, combining TADF assistant hosts with broad emission profiles and MRTADF emitters represents a key strategy in designing efficient and stable EMLs. In 2020, Chan et al. successfully fabricated efficient, stable, and narrow-band emission pure blue TADF-OLEDs by using a stable sky-blue TADF assistant host, HDT-1, to sensitize the pure blue MRTADF emitter v-DABNA[80]. The broad emission of HDT-1 provided significant spectral overlap between its PL spectrum edge and the absorption spectrum of v-DABNA. The high singlet and triplet energies of HDT-1 enable effective sensitization of the pure blue emitter v-DABNA. Additionally, HDT-1, with a high kRISC value of 8.6 × 105 s-1, effectively harvests triplet excitons and reduces the generation of long-lived triplet excitons on the MR-TADF emitter. Therefore, the two-unit stacked tandem hyperfluorescence OLED achieved a pure blue OLED with CIE coordinates of (0.13, 0.16), a high EQEmax of 41% (The EQE is of 32% at 1,000 cd/m2) and a LT95 of 18 hours at 1,000 cd/m2.
In 2024, Huang et al. perdeuterated the stable sky-blue TADF molecules 5CzBN and 5tCzBN, synthesizing D-5CzBN and D-5tCzBN[81]. The perdeuteration suppresses the non-radiative decay in TADF molecules, contributing to the high PLQY of D-5CzBN and D-5tCzBN (0.87 and 0.95, respectively). Additionally, perdeuteration resulted in slight blue shifted, which facilitated the efficient FET process when these perdeuterated TADF emitters were used as assistant hosts for pure blue emission. As a result, pure blue OLEDs incorporating D-5CzBN and D-5tCzBN as sky-blue assistant hosts and v-DABNA as the emitter achieved high EQEmax of 29.2% and 33.1%, with low efficiency roll-offs (24.1% and 29.0% of EQE at 1,000 cd/m2) and 398 hours and 1,365 hours of LT80 at 1,000 cd/m2.
Recently, Huang et al. synthesized three sky-blue TADF emitters 4CzBN-PhCN, 4tCzBN-PhCN and 4tCzBN-TPTRZ by introducing benzonitrile (PhCN) and 2,4,6-triphenyl-1,3,5-triazine (TPTRZ) as auxiliary acceptor groups into multi-carbazole-benzonitrile (CzBN) compounds, which exhibit both high stability under negative polaron and triplet exciton conditions and the fast RISC process[82]. Considering the high electroluminescent performance of 4CzBN-PhCN and 4tCzBN-PhCN (Discussed in Section 3.4.5), these two sky-blue TADF materials were employed as assistant hosts for the pure blue OLED based on the terminal emitter t-BuCz-DABNA. These devices achieved EQEmax of 30.8% and 27.8%, with EQE of 20.0% and 19.4% at 5,000 cd/m2. Notably, LT95 of 221 and 454 hours at 1,000 cd/m2 were reported, representing one of the most advanced results for blue devices in terms of lifetime.
Furthermore, incorporating hosts with low triplet energy and aligning the energy levels of the host, TADF assistant host, and MR emitter have been demonstrated as an effective approach for high-performance, long-lifetime blue OLEDs. In 2021, Jeon et al. proposed a triplet exciton recycling strategy for deep blue TADF-OLEDs and fabricated a triplet-exciton-distributed TADF (TED-TADF) device by doping a distributing TADF material and a MR-TADF emitter into a stable low triplet energy mixed host[83]. A host with a low triplet energy level, positioned between the distributing TADF material and the MR-TADF emitter in terms of triplet energy, can recycle the triplet excitons of distributing TADF material that are quenched by the host. Additionally, the MR-TADF emitter, having the lowest singlet energy, efficiently harvests the singlet excitons. Based on this device design, 9-(4-(4,6-diphenyl-1,3,5-triazin-2-yl)phenyl)-3,6-diphenyl-9H-carbazole (PCz-Trz) and 9-(5'-(4,6-diphenyl-1,3,5-triazin-2-yl)-[1,1':3',1''-terphenyl]-2'-yl)-3,6-diphenyl-9H-carbazole (PPCz-Trz) were synthesized as distributing TADF materials, with the low triplet energy level 2,2'-di(9H-carbazol-9-yl)-1,1'-biphenyl (oCBP): 9-(3'-(9Hcarbazol-9-yl)-5-cyano-[1,1'-biphenyl]-3-yl)-9H-carbazole-3-carbonitrile (CNmCBPCN) (50:50) serving as the mixed host, and v-DABNA acting as the deep-blue TADF emitter. Notably, the bottom-emission device based on PCzTrz achieved a high EQEmax of 33.5% and an estimated LT50 of 9,500 hours at 100 cd/m2, with a peak wavelength of 473 nm and a FWHM of 19 nm. The optimized top-emission device exhibited a high CCE of 432 cd/A, an EQEmax of 34.4%, an estimated LT50 of 6,100 hours at 100 cd/m2, and a deep blue emission with a CIEy value of 0.09.
A stable EML with high EUE can also be achieved by combining a TADF assistant host with high kRISC and a blue fluorescent emitter with high kr. In 2022, Lee et al. designed and synthesized a non-TADF-character blue MR emitter (CzBNPyr) and utilized it as the terminal emitter in hyperfluorescent (HF) OLEDs[84]. The DET process between TADF assistant host HDT-1 and MR emitter enables the quick conversion of long-lived exciton into the T1 of the MR emitter, and followed by a non-radiative ground state transition process, the reduced triplet exciton concentration facilitates improving device stability. Furthermore, the RISC process in the TADF assistant host generates additional singlet excitons, which can be efficiently transferred to the S1 of MR emitter for emission via FRET process, thereby high device efficiency is possible. As a result, the optimized blue HF-OLED device structure achieved an EQEmax of 19.4% and a LT90 of 69.2 hours at 1,000 cd/m2.
3.4.4 Phosphorescent assistant host
Phosphorescent materials as assistant hosts also demonstrate significant potential to improve exciton utilization and regulate the exciton dynamic. However, the development of phosphorescent assistant hosts for high-performance, long-lifetime blue OLEDs has been relatively slow, constrained by the challenges in designing and synthesizing deep blue phosphorescent materials[85]. Interestingly, several studies have reported that an efficient energy transfer process can still occur despite a small emission energy difference between the phosphorescent assistant host and the blue fluorescent emitter. The chemical structures of phosphorescent assistant hosts and emitters are shown in Figure 13. The performances of devices based on phosphorescent assistant hosts are shown in Table 5.
Device structure | Von (V) | EQEmax (%) | Lifetime (h) | CIE (x,y) | Ref. |
ITO/HATCN/NPD/Tris-PCz/mCBP: 20 wt% PtNON/mCBP: 2 wt% TBPe/mCBP: 10 wt% PtNON/mCBP: 2 wt% TBPe/mCBP: 10 wt% PtNON/mCBP: 2 wt% TBPe/mCBP: 6 wt% PtNON/mCBT/BPyTP/LiF/Al | - | 16.9 | LT70 = 602(1,000 nit) | (0.16, 0.25) | [45] |
ITO/HATCN/NPD/Tris-PCz/mCBP: 20 wt% PtNON/mCBP: 10 wt% PtNON/mCBP: 2 wt% TBPe/mCBP: 10 wt% PtNON/mCBP: 6 wt% PtNON/mCBT/BPyTP/LiF/Al | - | 18.0 | LT70 = 761(1,000 nit) | (0.16, 0.29) | [45] |
ITO/mCBP: 6 wt% Re2O7/m-CBP/m-CBP: TSPO1: 5 wt% (dfpysipy)2Ir(mpic): 1 wt% TBPe/TSPO1/Rb2CO3/Al | 3.6 | 15.3 | LT50 = 0.89(400 nit) | (0.14, 0.19) | [86] |
ITO/BCFN: 5 wt% NDP9/BCFN/Ir(dpbic)3/Ir(dpbic)3: SiDBFCz: Ir(cb)3 (50:50:10)/SiDBFCz/TBPi/Liq/Mg:Ag(9:1) | 3.3 | 14.4 | LT80 = 253(500 nit) | (0.15, 0.17) | [88] |
ITO/BPBPA: 30 wt% HATCN/BPBPA/mCBP/mCBP: SiCz2Trz: 20 wt% CN-Ir: 0.5 wt% v-DABNA/DBFTrz/ZADN/LiF/Al | 3.0 | 27.3 | LT50 = 121(1,000 nit) | (0.132, 0.162) | [92] |
ITO/HATCN/TAPC/mCBP: 15 wt% f-CF3 : 3 wt% t-DABNA/TmPyPb/LiF/Al | 5.2 | 23.8 | LT50 = 0.27(1,000 nit) | (0.13, 0.14) | [93] |
ITO/HATCN/TAPC/mCBP: 15 wt% f-PhCF3 : 2 wt% 2TCzBN/TmPyPb/LiF/Al | 5.4 | 24.0 | LT50 = 0.25(1,000 nit) | (0.11, 0.36) | [93] |
ITO/HATCN/TAPC/TCTA/mCBP/DPEPO: 8 wt% Pt-Adaph: 3 wt% mBP-DABNA-Me/DPEPO/TmPyPB/LiF/Al | 3.66 | 25.0 | - | (0.13, 0.17) | [94] |
EL: electroluminescence; OLEDs: organic light-emitting diodes; EQE: external quantum efficiency; CIE: Commission Internationale de l'Eclairage.
In 2018, Ahn et al. used blue phosphorescent assistant host bis[2',6'-difluoro-4-(trimethylsilyl)-2,3'-bipyridine]iridium(III) (4-methylpyridinepicolinate) ((dfpysipy)2Ir(mpic)) to sensitize blue fluorescent emitter 2,5,8,11-Tetra-tert-butylperylene (TPBe) within a mixed host[86]. Despite the emission energy between the phosphorescent assistant host and the fluorescent emitter was only 0.05 eV, the large overlap between the PL emission of phosphorescent assistant host and the absorption of TPBe support the occurrence of efficient FRET process. As a result, the blue device achieved a high EQEmax of 15.3%, which is 2.2 times higher than that of the non-sensitized device. Furthermore, the lifetime of the sensitized device was 10 times longer than that of the non-sensitized device. However, limited by the instability of the host material, the LT50 of the sensitized device was only 0.89 hour at 400 cd/m2.
Simultaneously, Heimel et al. proposed a unicolored phosphorescent-sensitized fluorescence (UPSF) strategy[87]. They co-doped a blue phosphorescent emitter and a blue fluorescent emitter with similar emission spectra into the host, and fabricate devices with a triplet-singlet dual emission system. The device structure with energy levels, and molecular structures of functional materials, are shown in Figure 14a. The rapid FET process from the blue phosphorescent assistant host to the blue fluorescent emitter reduced the radiative decay time of the blue phosphorescent assistant host. However, the increased doping concentration of the blue fluorescent emitter reduces the distance between the phosphorescent and fluorescent emitters, which facilitates the DET process, leading to the quenching of triplet excitons by the fluorescent emitter (Figure 14b). Additionally, benefiting from the shortened radiative decay time of triplet excitons, the accumulation of triplet excitons is suppressed, leading to an extension of device lifetime as the doping concentration of the fluorescent emitter increases (Figure 14c). Notably, the UPSF device with a phosphorescent assistant host to fluorescent emitter doping ratio of 20:1.5 achieved a high EQE of 10.5%, an estimated LT80 of 320 hours at 1,000 cd/m2, and a sky-blue emission with CIE coordinates of (0.139, 0.274). Therefore, it is necessary to suppress DET process between the phosphorescent assistant hosts and the blue fluorescent emitters for high-performance blue OLED.
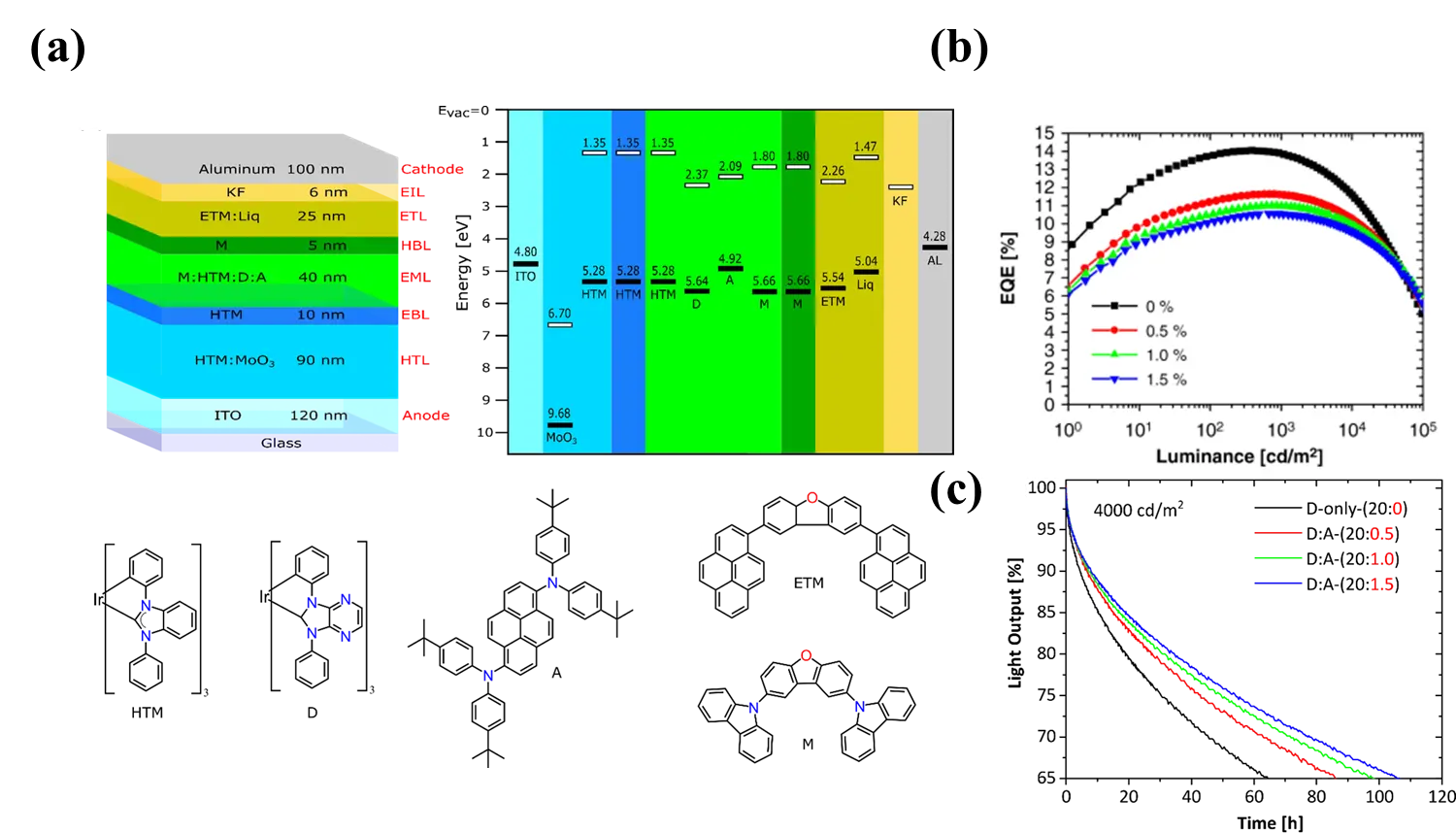
Figure 14. (a) Device structure with energy levels and molecular structures of materials used in the device; (b) EQE vs luminance; (c) lifetime measurement of UPSF devices with varying emitter concentrations at 4,000 cd/m2. Republished with permission from[87]. EQE: external quantum efficiency; UPSF: unicolored phosphorescent-sensitized fluorescence.
In 2019, Klimes et al. inhibited the DET process by separating the blue phosphorescent assistant host PtNON and the fluorescent emitter TPBe within a step-wise graded-doped EML structure[45]. The EML structure with independently doped layers increased the distance between PtNON and TPBe, which inhibited the DET process. In addition, the step-wise graded-doped of the EML moved the charge recombination zone away from the EBL and broadened the exciton formation region, thereby enhancing both the efficiency and lifetime of the blue fluorescent OLED. Consequently, the device achieved a high EQEmax of over 15%, an estimated LT70 of more than 600 hours at 1,000 cd/m2, and blue emission with CIE coordinates of (0.16, 0.25).
For blue phosphorescent OLED, the slow DET process of long-lived triplet excitons from the host to the phosphorescent emitter may lead to a high concentration of triplet excitons on the host. In 2022, Kim et al. introduced an Ir-based intermediate energy carrier (IEC) into the EML, creating multiple energy transfer pathways between the host, IEC and phosphorescent emitter[88]. They used 9-(4-(triphenylsilyl)dibenzo[b,d]furan-2-yl)-9H-carbazole (SiDBFCz) as the EML host, fac-tris(1,3-diphenyl-benzimidazolin-2-ylidene-C,C2')iridium(III) (Ir(dpbic)3) as the IEC, and Ir(cb)3 as the blue phosphorescent emitter. The extra DET process from the host to the IEC depletes long-lived T2 excitons on the host, while the T1 excitons on the IEC are efficiently transferred to Ir(cb)3 via DET and rapid FET processes. Therefore, the blue phosphorescent OLED with an IEC achieved a high EQEmax of 14.4% an estimated LT50 of 13,830 hours at 100 cd/m2, with a CIEy value of 0.17.
Benefiting from the small Stokes shift of MRTADF materials, blue OLEDs that use MRTADF materials as the terminal emitters mitigate the need for deep-blue phosphorescent assistant hosts. Therefore, introducing phosphorescent assistant hosts in blue MR-TADF-OLEDs has been demonstrated as a feasible strategy for high-performance blue OLEDs[89-91]. In 2021, Chung et al. developed an energy-exchanging phosphorescence and TADF (EPHTADF) device using the phosphorescent assistant host fac-tris(1-(2,6-diisopropylphenyl)-2-(3-cyanophenyl)-1H-imidazolyl)iridium(III) (CN-Ir) and the MR-TADF emitter v-DABNA with similar emission energies[92]. The exciton management strategy of the device is shown in Figure 15a. The triplet energy on CN-Ir is transferred to v-DABNA through FRET and DET processes, which shortens the triplet exciton lifetime of CN-Ir. Additionally, there was a reverse DET from the singlet excitons of v-DABNA back to the triplet excitons of CN-Ir, which further reduced the generation of long-lived triplet excitons from the MR-TADF emitter. The additional energy transfer pathway reduces long-lived triplet excitons and shortens the triplet exciton lifetime. Thus, the blue EPHTADF device, compared to the device without a phosphorescent assistant host, EQEmax was increased from 19.5% to 27.3%, LT50 at 1,000 cd/m2 from 26 hours was extended to 121 hours (Figure 15b,c). In addition, the top-emitting device based on this strategy achieved excellent performance with a high CE of 37 cd/A, a CCE of 430 cd/A, a deep blue CIEy value of 0.086, and an estimated LT50 of 31,130 hours at 100 cd/m2.
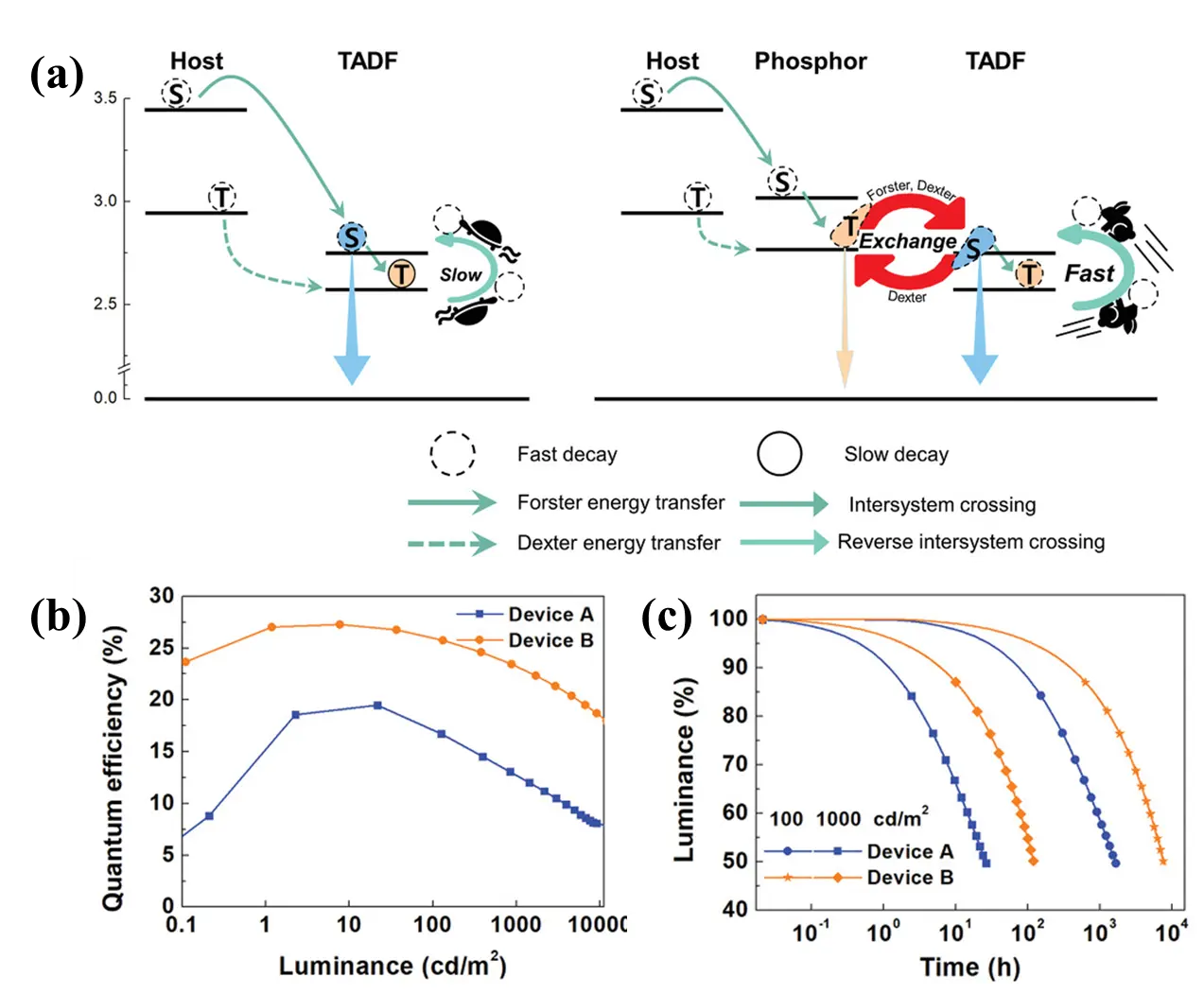
Figure 15. (a) Emission mechanism of conventional TADF devices and EPHTADF devices; (b) EQE-luminance curves; (c) device lifetime curves at 1,000 cd/m2 and 100 cd/m2. The EML of Device A consists of 1% v-DABNA doped in mCBP: SiCz2Trz, while the EML of Device B consists of 0.5% v-DABNA and 20% CN-Ir doped in mCBP: SiCz2Trz. Republished with permission[92]. TADF: thermally activated delayed fluorescence; EQE: external quantum efficiency; EPHTADF: energy-exchanging phosphorescence and TADF; EML: emission layer; mCBP: 3,3'-di(9H-carbazol-9-yl)-1,1'-biphenyl.
In 2022, Qin et al. designed the blue phosphorescent emitter f-CF3 and the blue-green phosphorescent emitter f-PhCF3[93]. Benefiting from high PLQYs and short radiative lifetimes, the blue phosphorescent devices based on f-CF3 and f-PhCF3 achieved EQEmax of 10.4% and 12.8%, respectively, with emission peaks at 478 nm (blue) and 495 nm (bluish-green). Furthermore, f-CF3 and f-PhCF3 were employed as the assistant hosts for the MRTADF emitters t-DABNA and 2TCzBN to further enhance triplet exciton utilization and color purity. Specifically, the blue OLEDs based on t-DABNA and 2TCzBN achieved EQEmax of 23.8% and 24.0%, respectively, while maintaining EL spectra similar to non-sensitized MRTADF devices. However, due to the inherent instability of the phosphorescent emitter and the lack of a tailored device structure, the MRTADF devices sensitized by f-CF3 and f-PhCF3 achieved LT50 of 0.27 hours and 0.25 hours at 1,000 cd/m2. Nevertheless, the lifetime of sensitized devices is nearly twice that of non-sensitized devices.
In 2024, Baek et al. developed a blue OLED with EQEmax of 25.0% and a peak intensity at 465 nm, using Pt-Adaph as a phosphorescent assistant host for the blue MRTADF emitter mBP-DABNA-Me[94]. They discovered that the careful combination of the phosphorescent assistant host and the MRTADF emitter significantly enhanced horizontal emitting dipole orientation to 81%, thereby improving light outcoupling. The square planar flat structures of the assistant host and emitter, along with their enhanced van der Waals interactions, promoted the parallel alignment of the transition dipole moments of two molecules. Additionally, the energy transfer process from the phosphorescent assistant host to the MRTADF emitter efficiently managed the distribution of triplet excitons, reducing efficiency roll-off in the blue OLED, with an EQE of 20.9% at 300 cd/m2.
3.4.5 Exciplex and electroplex hosts
As mentioned above, traditional fluorescent type mixed host can effectively regulate carrier balance, thereby broadening the carrier recombination zone, which is a crucial strategy for high-performance blue OLEDs. However, in a mixed-host EML, emission occurs through carrier trapping by the emitter, which is prone to device degradation caused by TPA process. Specifically, carrier trapping and accumulation are key factors that contribute to the promotion of the TPA process[10]. By adjusting the ratio of the mixed host, the balanced charge transport can be achieved, helping to reduces excess polarons. However, compared to the hosts, the emitters with a smaller energy gap is more capable of accommodating charges, thereby the enhanced charge capture by the emitter[95]. Even at lower doping concentrations, the emitters can maintain a charge density comparable to that of the hosts[96]. As a result, the high carrier density on the emitter leads to severe TPA process, the defects generated from the TPA process play an important role in the degradation of OLED devices[95,97,98]. For instance, in 2022, Yang et al. investigated the impact of the electrical properties of a cohost EML on the degradation of blue phosphorescent organic light-emitting diodes (PHOLEDs)[95]. The results indicated that the TPA process induced by a high carrier density on the dopant is the primary degradation mechanism, the generation of defects from the TPA process become a main cause of short device lifetime. Additionally, the distribution of excitons and defects in cohost devices with different ratios revealed that the lifetime of blue PHOLEDs depends not only on the total defect density but also on the spatial distribution of excitons and defects within the EML. Furthermore, the defects generated by the TPA process are also a major factor contributing to the degradation of blue TADF-OLEDs[97,98]. Therefore, minimizing carrier density on the emitters and regulating the uniform distribution of excitons and defects within the EML can suppress exciton quenching and extend the lifetime of blue OLEDs.
A blue OLED based on an exciplex-type mixed host achieves emission through energy transfer from the mixed host to the emitter, effectively suppressing the severe TPA process caused by charge trapping[99]. In an exciplex host, electrons are transported through the n-type host and holes through the p-type host, leading to the separation of electrons and holes and the formation of excitons between n-type host and p-type host[100]. Similar to traditional fluorescent type mixed host, charge transport balance can be achieved by adjusting the ratio of hosts, thereby broadening the emission zone. Additionally, the naturally separated HOMO and LUMO distributions confer TADF properties to the exciplex, leading to higher EUE[101,102]. Despite the advantages of exciplexes, when used as hosts for blue or deep-blue emitters, exciplexes should exhibit high triplet energy to ensure efficient energy transfer. However, the strong binding between n-type and p-type hosts typically results in low triplet energy of the exciplex, which hinders the development of blue or deep-blue OLEDs based on exciplex hosts[1,101]. The chemical structures of n-type hosts and p-type hosts for exciplex are shown in Figure 16 and Figure 17. The performances of devices based on exciplex hosts are shown in Table 6.
Device structure | Von (V) | EQEmax (%) | Lifetime (h) | CIE (x,y) | Ref. |
ITO/HATCN/BCFN/SiCzCz/SiCzCz: SiTrzCz2: D-5CzBN (55:30:15)/mSiTRZ/DPPyA: Liq/LiF/Al | - | 29.0 | LT80 = 210(1,000 nit) | (0.16, 0.24) | [81] |
ITO/HATCN/BCFN/SiCzCz/SiCzCz: SiTrzCz2: D-5tCzBN (55:30:15)/mSiTRZ/DPPyA: Liq/LiF/Al | - | 29.2 | LT80 = 949(1,000 nit) | (0.17, 0.28) | [81] |
ITO/HATCN/BCFN/SiCzCz/SiCzCz: SiTrzCz2: 4CzBN-PhCN (48:32:20)/SiTrzCz2/DPPyA: Liq/LiF/Al | - | 28.0 | LT95 = 26.7(5,000 nit) | (0.21, 0.40) | [82] |
ITO/HATCN/BCFN/SiCzCz/SiCzCz: SiTrzCz2: 4tCzBN-PhCN (48:32:20)/SiTrzCz2 /DPPyA: Liq/LiF/Al | - | 37.1 | LT95 = 37.6(5,000 nit) | (0.23, 0.48) | [82] |
ITO/HATCN/BCFN/SiCzCz/SiCzCz: SiTrzCz2: 4tCzBN-TPTRZ (48:32:20)/SiTrzCz2/DPPyA: Liq/LiF/Al | - | 27.9 | LT95 = 16.4(5,000 nit) | (0.23, 0.44) | [82] |
ITO/BPBPA: 30 wt% HATCN/BPBPA/PCZAC/oCBP: mSiTrz: 20 wt% Ir(cb)3/DBFTrz/ZADN/LiF/Al | 2.5 | 21.6 | LT50 = 1,900(100 nit) | (0.14, 0.16) | [103] |
ITO/BPBPA: 30 wt% HATCN/BPBPA/PCZAC/oCBP:pSiTrz: 20 wt% Ir(cb)3/DBFTrz/ZADN/LiF/Al | 2.5 | 19.1 | LT50 = 1,900(100 nit) | (0.14, 0.16) | [103] |
ITO/BPBPA: 30 wt% HATCN/BPBPA/mCBP/mCBP: mSiTrz-mCN: 20 wt% Ir(cb)3/DBFTrz/ZADN/LiF/Al | 3.6 | 21.0 | LT50 = 3,130(100 nit) | (0.14, 0.18) | [104] |
ITO/HAT-CN/BCFN/SiCzCz/SiTrzCz2: SiCzCz: BD-02 (0.60:0.27:0.13)/mSiTrz /mSiTrz: Liq (5:5)/LiF/Al | 2.8 | 25.4 | LT70 = 1,113(1,000 nit) | (0.141, 0.197) | [106] |
ITO/HATCN/BCFN/SiCzCz/SiCzCz: SiTrzCz2: PtON-TBBI: TBE01 (65:35:13:0.4)/2SiTrzPh/2SiTrzPh: Liq(5:5)/LiF/Al | - | 25.4 | LT95 = 42.3(1,000 nit) | (-, 0.165) | [107] |
ITO/HATCN/BCFN/SiCzCz/SiCzCz: SiTrzCz2: PtON-TBBI: TBE02 (65:35:13:0.4)/2SiTrzPh/2SiTrzPh: Liq(5:5)/LiF/Al | - | 25.8 | LT95 = 72.9(1,000 nit) | (-, 0.165) | [107] |
ITO/HAT-CN/Tris-PCz/Tris-PCz: 3CzTRZ: v-DABNA (50:50:1)/SF3-TRZ/SF3-TRZ: 50 wt% Liq/Liq/Al | - | 19.0 | LT50 = 300(1,260 nit) | (-, 0.36) | [108] |
ITO/HATCN/F6-TCNNQ/TAPC/TCTA/mCP/p-PhBCzPh: PO-T2T: DspiroAc-TRZ: 2TCzBN (50:20:30:1)/PPF/TmPyPb/LiF/Al | 2.5 | 36.2 | LT80 = 4.8(1,000 nit) | (0.11, 0.39) | [109] |
ITO/DNTPD/BPBPA/PCZAC/mCBP: DBFTrz: Ir(CNpi)3 (50:50:5)/DBFTrz/ZADN/LiF/Al | - | 18.0 | LT50 = 38.9(500 nit) | (0.16, 0.29) | [110] |
Ag/ITO/BPBPA: 30 wt% HATCN/BPBPA/mCBP/mCBP: SiCzTrz: Ir(cb)3 (50:50:20)/DBFTrz/ZADN/Liq/Ag:Mg | - | 27.6 | LT50 = 10,700(100 nit) | (0.12, 0.13) | [111] |
ITO/BPBPA: 30 wt% HATCN/BPBPA/mCBP/mCBP: SiTCNCz: Ir(cb)3 (50:50:20)/DBFTrz/ZADN/LiF/Al | 2.8 | 20.4 | LT50 = 3,380(100 nit) | (0.14, 0.18) | [112] |
EL: electroluminescence; OLEDs: organic light-emitting diodes; EQE: external quantum efficiency; CIE: Commission Internationale de l'Eclairage.
Introducing bulky blocking groups into the host material to weaken the binding between n-type and p-type hosts is a common strategy for achieving high triplet energy exciplexes. In 2019, Choi et al. synthesized two n-type hosts, 2-phenyl-4,6-bis(4-(triphenylsilyl)phenyl)-1,3,5-triazine (pSiTrz) and 2-phenyl-4,6-bis(3-(triphenylsilyl)phenyl)-1,3,5-triazine (mSiTrz), with high triplet energy using triphenylsilyl blocking groups[103]. Combined with the p-type host oCBP, the exciplexes pSiTrz: oCBP and mSiTrz: oCBP exhibited high triplet energy exceeding 2.90 eV. As a result, the efficient energy transfer enabled blue phosphorescent OLEDs based on Ir(cb)3 to achieve EQEmax of 21.6% and 19.1%, respectively. Additionally, balanced charge transport reduced efficiency roll-off, with EQEs of 21.1% and 18.6% at 1000 cd/m2. Notably, both devices achieved LT50 of 1,900 hours at 100 cd/m2 with CIE coordinates of (0.14, 0.16).
Furthermore, in 2021, they synthesized three advanced n-type hosts: 2-(4,6-bis(3-(triphenylsilyl)phenyl)-1,3,5-triazin-2-yl)benzonitrile (mSiTrz-oCN), 3-(4,6-bis(3-(triphenylsilyl)phenyl)-1,3,5-triazin-2-yl)benzonitrile (mSiTrz-mCN), and 4-(4,6-bis(3-(triphenylsilyl)phenyl)-1,3,5-triazin-2-yl)benzonitrile (mSiTrz-pCN)[104]. These n-type hosts were combined with the p-type host mCBP in a 50:50 ratio to form three exciplex hosts. The devices were fabricated with architecture of: ITO/BPBPA: 30 wt% HATCN/BPBPA/mCBP/mCBP: n-type host: 20 wt% Ir(cb)3/DBFTrz/ZADN/LiF/Al. Among these devices, exciplex host mSiTrz-mCN: mCBP with higher triplet energy, which ensured efficient energy transfer from the host to the emitter. Consequently, it exhibited the best performance for blue phosphorescent OLEDs, achieving a high EQEmax of 21.0%, a LT50 of 3,130 hours at 100 cd/m2, with CIE coordinates of (0.14, 0.18).
In 2022, Kim et al. synthesized four p-type hosts (PH1, PH2, PH3, and PH4) and one n-type host PH5 with high T1 levels and strong BDE using triphenylsilyl and 1,3,5-triazine as bulky blocking groups[105]. They combined the four p-type hosts with the n-type host to fabricate four blue phosphorescent OLEDs with different charge behaviors, using Firpic as the emitter. Balanced charge transport and reduced carrier trapping contributed to the high performance of these blue phosphorescent OLEDs. Notably, the blue OLED based on the exciplex host PH3: PH5 achieved a high EQEmax of 20.1%, low efficiency roll-off (13.3% of EQE at 10,000 cd/m2), and a LT50 of 51 hours at 500 cd/m2.
In addition to high triplet energy levels and strong BDE, exciplexes with obvious TADF characteristics can further improve blue OLED performance. In 2022, Sun et al. synthesized a stable blue phosphorescent molecule, PtON-TBBI (BD-02)[106]. To maximize the stability of devices based on BD-02, they also developed high triplet-energy stable silicon-based n-type host 9-(3-(triphenylsilyl)phenyl)-9H-3,9'-bicarbazole (SiCzCz) and p-type host 9,9'-(6-(3-(triphenylsilyl)phenyl)-1,3,5-triazine-2,4-diyl)bis(9H-carbazole) (SiTrzCz2), which were used to create an exciplex host SiCzCz: SiTrzCz2 for blue phosphorescent OLEDs. The RISC process of the exciplex host effectively harvest triplet excitons, and efficient energy transfer from the host to the emitter gives a high EQEmax of 25.4%. The exciplex host promoted charge balance within the device, broadened the emission region and suppressed TTA and TPA process, which contributes to an excellent device lifetime. Impressively, blue phosphorescent OLED achieved a LT70 of 1,113 hours at 1,000 cd/m2, with a CIEy value of 0.197.
Due to its high PLQY (54%), high triplet energy levels, and intrinsic material stability[106], the blue exciplex SiCzCz: SiTrzCz2 is commonly utilized in high-performance and long-lifetime blue OLEDs. In 2022, Kim et al. designed and synthesized two MR-TADF emitters, TBE01 and TBE02, for efficient and long-lifetime deep-blue TADF-OLEDs[107]. They utilized the exciplex host (SiCzCz: SiCzTrz2) and the phosphorescent assistant host PtON-TBBI to sensitize MR-TADF emitters. Specifically, singlet excitons from the exciplex host are transferred to the phosphorescent assistant host and emitter via FRET process. The large spectral overlap between the phosphorescent assistant host and the emitter facilitates efficient FRET process from the stable phosphorescent PtON-TBBI to the emitter, rather than DET process. Additionally, the emitters TBE01 and TBE02 have deep HOMO levels, which reduce hole trapping and polaron formation in the emitter. Moreover, two MR-TADF emitters with large k RISC can effectively reduce the number of triplet excitons on the emitter. The optimized bottom-emitting device structure is shown in Figure 18a. As a result, the devices based on TBE1 and TBE2 achieved EQEs of 25.4% and 25.8%, with LT95 of 42.3 hours and 72.9 hours at 1,000 cd/m2, respectively (Figure 18b,c,d). Additionally, the top-emitting device achieved a CCE of 441.4 cd/A, a LT50 of 681 hours at 1,260 cd/m2, and deep blue emission with a CIEy value of 0.058.
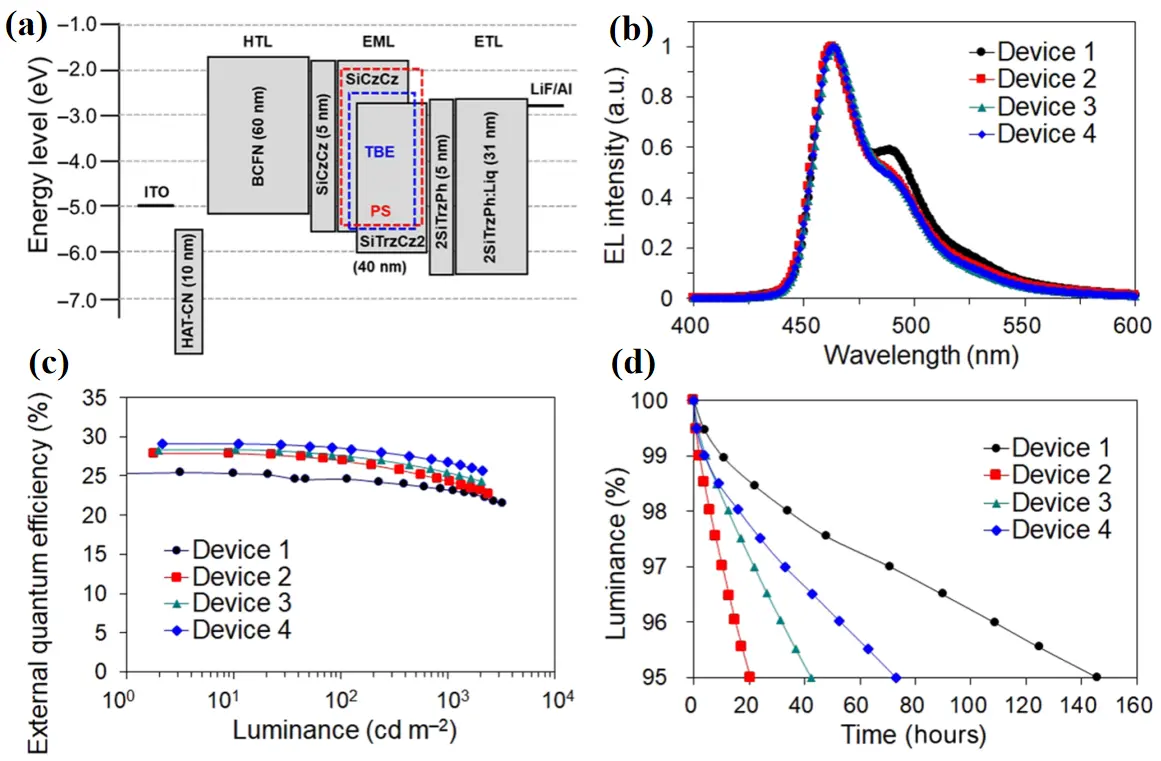
Figure 18. (a) Multiple-sensitized device structure with energy levels; (b) EL spectra; (c) EQE versus luminance plots; (d) luminance versus time plots for the following devices: PS only, PtON-TBBI (13 wt %); device 1, PtON-TBBI (13 wt %) + t-DABNA (0.4 wt %); device 2, PtONTBBI (13 wt %) + TBE1 (0.4 wt %); device 3, PtON-TBBI (13 wt %) + TBE2 (0.4 wt %); device 4. Republished with permission[107]. EQE: external quantum efficiency; EL: electroluminescence; HTL: hole-transport layer; EML: emission layer; ETL: electron transporting layer.
In 2024, Huang et al. utilized the stable blue exciplex SiCzCz: SiTrzCz2 as the host for sky-blue OLEDs based on perdeuterated TADF emitters D-5CzBN and D-5tCzBN[81]. These devices achieved high EQEmax of 29.0% and 29.2%, with low efficiency roll-offs (25.2% and 25.7% of EQE at 1,000 cd/m2) and 210 hours and 949 hours of LT80 at 1,000 cd/m2. The superior performance was attributed to the suppression of high-energy vibrations and the stable exciplex host.
As mentioned above, Huang et al. successfully designed and synthesized three highly efficient and stable sky-blue emitters 4CzBN-PhCN, 4tCzBN-PhCN and 4tCzBN-TPTRZ, by introducing rational auxiliary acceptor groups into CzBN-type TADF emitters[82]. The stable exciplex host SiCzCz: SiTrzCz2 was also utilized for device stability evaluation. Sky-blue OLEDs based on 4CzBN-PhCN, 4tCzBN-PhCN, and 4tCzBN-TPTRZ achieved EQEmax of 28.0%, 37.1%, and 27.9%, with EQE of 21.9%, 34.2%, and 24.5% at 5,000 cd/m2, respectively. Notably, these devices exhibited impressive LT95 of 26.7, 37.6, and 16.4 hours at 5,000 cd/m2.
In addition to designing and synthesizing efficient, stable exciplexes using traditional fluorescent type hosts, incorporating TADF emitters as the component of exciplex has also proven advantageous for constructing high-performance blue OLEDs. Nguyen et al. in 2020 combined the donor 9,9',9''-triphenyl-9H,9'H,9''H-3,3':6',3''-tercarbazole (Tris-PCz) with the TADF-type acceptors 3Cz-TRZ and BCz-TRZ to form exciplex, featuring dual RISC channels[108]. The triplet excitons generated directly on the TADF-type acceptor will be converted into singlet excitons through the RISC process, reducing the accumulation of triplet excitons on a single host and thereby inhibiting the damage to host molecules. Additionally, the RISC process in TADF materials enhances the utilization efficiency of triplet excitons. Therefore, devices based on exciplex emitters Tris-PCz: 3Cz-TRZ and Tris-PCz: BCz-TRZ achieved EQEmax of 8.9% and 11.9%, respectively, with LT50 of 337 hours and 292 hours at a current density of 3.3 mA/cm2. Additionally, the blue OLED using the exciplex Tris-PCz: 3Cz-TRZ as the host for the v-DABNA emitter achieved an EQEmax of 19% and a LT50 of over 300 hours at 1,260 cd/m2.
Furthermore, in 2024, Liu et al. developed a tricomponent exciplex p-PhBCzPh: PO-T2T: DspiroAc-TRZ, which featured multiple RISC channels and was used as the host for high-performance blue OLEDs[109]. There are three RISC channels in p-PhBCzPh: PO-T2T (exciplex-1), DspiroAc-TRZ: PO-T2T (exciplex-2), and DspiroAc-TRZ (TADF). These RISC channels accelerated the upconversion of non-radiative triplet excitons, which increased the utilization of triplet excitons and reduced the concentration of long-lived triplet excitons. Subsequently, the singlet energy on the tricomponent exciplex host was exothermically transferred to the MRTADF emitter 2TCzBN with a high radiative transition rate. Consequently, the blue MRTADF-OLED based on the optimized tricomponent exciplex host achieved EQEmax of 36.2%, low efficiency roll-off (EQE of 26.1% at 1,000 cd/m2), and a LT80 of 4.8 hours at 1,000 cd/m2. Although the lifetime of device was limited by the stability of the exciplex components, the superior triplet exciton management lead to a lifetime more than three times longer than that of device based on traditional binary exciplex host.
In addition to exciplexes, electroplexes formed through weak binding between mixed hosts have also been demonstrated as an effective host strategy for high-performance blue OLEDs. In 2017, Song et al. proposed the concept of an electroplex host[110]. Unlike exciplexes, the weak binding in electroplex results in low exciton binding energy, thereby preserving high emission energy. The higher triplet energy makes electroplex more suitable as hosts for blue OLED. Furthermore, the emission mechanism of OLEDs based on electroplex hosts is similar to that of exciplexes, relying on energy transfer rather than charge trapping by the emitter. Therefore, they developed an electroplex mCBP: DBFTrz (2,8-bis(4,6-diphenyl-1,3,5-triazin-2-yl)dibenzo[b,d]furan) and was used as the host for the phosphorescent emitter fac-tris(3-(1-(2,6-diisopropylphenyl)-1H-imidazol-2-yl)benzonitrile) iridium (Ir(CNpi)3), achieving an EQEmax of 18.0% and a LT50 of 38.9 hours at 500 cd/m2.
To advance the application of electroplexes in high-performance pure blue OLEDs, in 2019, Jung et al. designed and synthesized a stable n-type host 9-(4-phenyl-6-(3-(triphenylsilyl)phenyl)-1,3,5-triazin-2-yl)-9H-carbazole (SiCzTrz) with high triplet energy and developed the electroplex host SiCzTrz: mCBP for pure blue OLEDs[111]. Using SiCzTrz: mCBP as the host and Ir(cb)3 as the pure blue phosphorescent emitter, the top-emission blue phosphorescent OLED achieved a high EQEmax of 27.6%, an estimated LT50 of 10,700 hours at 100 cd/m2, and pure blue emission with CIE coordinates of (0.12, 0.13).
Similar to exciplex hosts, incorporating TADF materials as components of electroplexes can also enhance the performance of blue devices. Therefore, in 2021, Yun et al. synthesized the n-type TADF host 3-(4-(9H-carbazol-9-yl)-6-(3-(triphenylsilyl)phenyl)-1,3,5-triazin-2-yl)benzonitrile (SiTCNCz) and developed an electroplex SiTCNCz: mCBP with dual RISC channels[112]. Blue phosphorescent OLED based on emitter Ir(cb) 3 achieved a high EQEmax of 20.4% and an estimated LT50 of 3,380 hours at 100 cd/m2, which is more than 2.6 times that of the blue phosphorescent OLED with a single RISC channel electroplex host.
3.5 High performance and long-lifetime solution-processed blue OLEDs
Currently, most commercial OLED panels are mass-produced using thermal evaporation technology. However, this method requires expensive evaporation equipment, and unsuitable for fabricating the large-scale flexible OLEDs[113]. In contrast, solution processing is a low-cost and large-area production technology for OLEDs fabrication. At present, many researches have demonstrated solution-processed blue OLEDs with EQE exceeding 20%[114-120]. However, the issue of lifetime for solution-processed blue OLEDs remains challenging and has been mentioned in limited studies[121-125]. Solution-processed EML tends to experience faster aggregation of emitter molecules under electrical stress, the aggravated TTA and TPA lead to more severe degradation in devices stability compared to evaporated EMLs[125,126]. Additionally, the efficiency and lifetime of solution-processed OLEDs lag far behind those of vacuum-evaporated OLEDs, which are attributed to the lack of suitable transport functional materials leads to the difficulties in managing charge transporting in solution processed OLEDs[113,126,127]. In addition to the internal degradation processes in devices, solution-processed OLEDs usually fail to achieve balanced charge transport and high exciton utilization efficiency, together with the poor film morphology and the increased defects in multi-layer mixing, which contribute to the lower device efficiency and shorter lifetimes[121,123]. Consequently, it is difficult to prepare high-performance, long-lifetime solution-processed blue OLEDs. Although the performance difference between solution-processed blue OLEDs and evaporated OLEDs still exists, the difference is expected to gradually close with the development of advanced solution-processable materials and optimized device designs. The chemical structures of materials for solution processed blue OLEDs are shown in Figure 19.
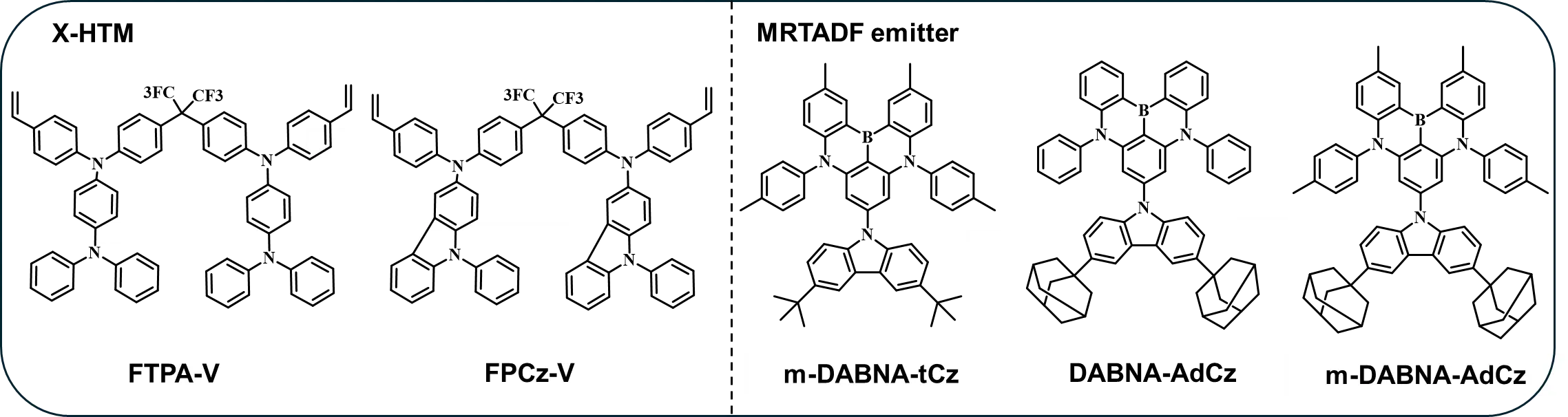
Figure 19. The chemical structures of X-HTMs and MRTADF emitters for solution processed blue OLEDs. HTMs: hole-transporting materials; MRTADF: multi-resonant thermally activated delayed fluorescent; OLEDs: organic light-emitting diodes.
In 2023, Liu et al. developed three solution-processable bipolar host materials with sequentially decreasing triplet energies: 3',5'-di(9Hcarbazol-9-yl)-2,6-dimethyl-[1,1'-biphenyl]-4-carbonitrile (DMBN-PDC), 3',4',5'-tri(9H-carbazol-9-yl)-2,6-dimethyl-[1,1'-biphenyl]-4-carbonitrile (DMBN-PTC), and 3',4',5'-tri(9H-carbazol-9-yl)-2,6-dimethyl-[1,1'-biphenyl]-4-carbonitrile) (MBN-PTC) for high-performance solution-processed blue OLED[123]. As shown in Figure 20a, DMBN-PTC with moderate T1 energy doped with a blue TADF emitter 5TCzBN at appropriated content exhibited the best charge balance and photochemical stability, which enables a uniformed triplet exciton distribution among host and guest to reduce exciton quenching. Accordingly, the optimized device achieved a high EQEmax of 20.8%, EQE of 20.1% at 1,000 cd/m2 and a LT50 of 398.3 hours at 500 cd/m2 (Figure 20b,c). Furthermore, incorporating the MR-TADF emitter 2TCzBN to improve exciton utilization for developing TADF-sensitized device, a higher EQEmax of 23.1%, with EQEs of 22.2% and 18.1% at 1,000 cd/m2 and 5,000 cd/m2, respectively, and extended device lifetime of 456.3 hours at 500cd/m2 were achieved.
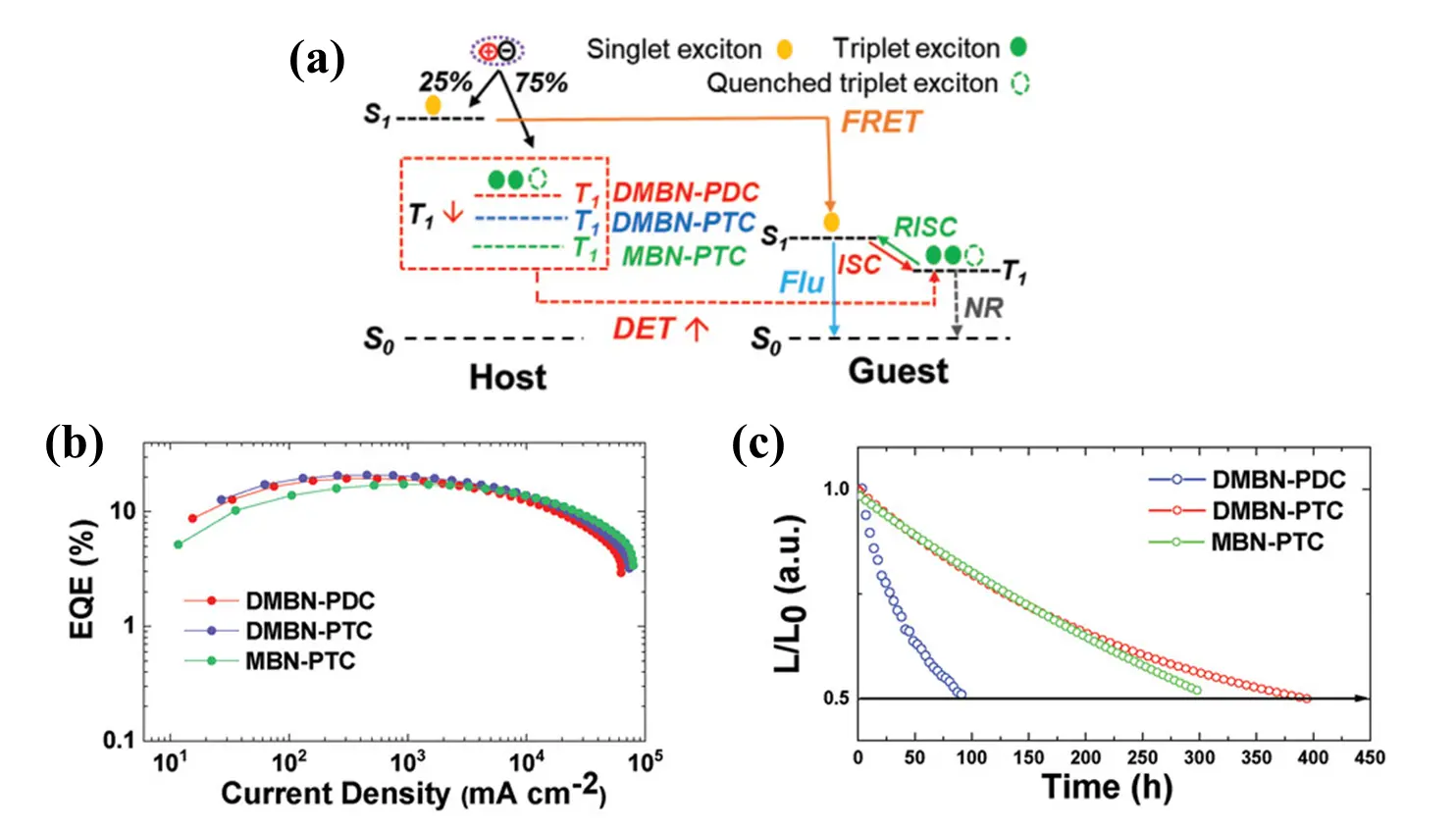
Figure 20. (a) The schematic diagram of the energy transfer process between the host and guest; (b) EQE-current density curves; (c) operational lifetime of the devices measured at the initial luminance of 500 cd/m2. Republished with permission[123]. EQE: external quantum efficiency.
In 2024, Zhang et al. developed two cross-linkable hole transport materials (X-HTMs), N1,N1'-((perfluoropropane-2,2-diyl)bis(4,1-phenylene))bis(N4,N4- diphenylN1-(4-vinylphenyl)benzene-1,4-diamine) (FTPA-V) and N,N'-((perfluoropropane-2,2-diyl)bis(4,1-phenylene))bis(9-phenyl-N-(4-vinylphenyl)-9H-carbazol-3-amine) (FPCz-V), specifically designed for solution-processed blue OLEDs based on MRTADF emitter M-t-DABNA and compared them with the commonly used solution-processed HTM TFB (poly-(9,9-dioctylfluorene-alt-N-(4-sec-butylphenyl)-diphenylamine))[124]. Compared to TFB-based devices, those incorporating X-HTMs exhibited better energy level alignment, superior film morphology, and improved solvent resistance, which enhanced interfacial contact between the X-HTMs and adjacent functional layers. Specifically, the matching energy levels facilitated efficient hole injection, the smooth film morphology reduced defect density at the interfaces, and the stronger solvent resistance of X-HTMs minimized interface penetration and mixing, thereby preventing the introduction of traps in the EML that could degrade device performance[128]. Consequently, blue devices based on X-HTMs achieved higher performance, with EQEmax of 4.7% and 7.0% for FTPA-V and FPCz-V, respectively, were obviously superior to for the TFB-based device (EQE of 2.3%). Furthermore, X-HTMs exhibited higher triplet energy levels, stability, and balanced charge transport relative to TFB, reducing the formation of triplet excitons and polarons in the HTL. As a result, devices based on X-HTMs demonstrated longer operational lifetimes, with LT95 of 82 hours and 153 hours at 1,000 cd/m2 for FTPA-V and FPCz-V, respectively, compared to just 36 hours for the TFB-based device.
In addition to host and transport layer materials, the development of stable, solution-processable blue emitters is a critical prerequisite for long-lifetime solution-processed blue OLEDs. In 2024, Li et al. developed three deep blue MR-TADF emitters: DABNA-AdCz, m-DABNA-tCz, and m-DABNA-AdCz[125]. Notably, m-DABNA-AdCz with the methyl and adamantyl end-capped modification reduced vibrational coupling, thereby stabilizing the excited state. Additionally, the incorporation of the adamantyl group further enhanced the stability of the EML. As a result, the solution-processed blue OLED based on m-DABNA-AdCz achieved a CE of 6.0 cd/A and a LT95 of 55.7 hours at 1,000 cd/m2, with a FWHM of 21 nm and a CIEy of 0.076. The performances of solution processed blue devices are shown in Table 7. The EL performance for representative blue OLEDs is summarized in Table 8.
Device structure | Voltage (V) | EQEmax (%) | Lifetime (h) | CIE (x,y) | Ref. |
ITO/PEDOT: PSS/PVK/DMBN-PTC: 30 wt% 5TCzBN/T2T/NBphen/CsF/Al | 4.4 | 20.8 | LT50 = 398.3(500 nit) | (0.21, 0.44) | [123] |
ITO/PEDOT: PSS/PVK/DMBN-PTC: 30 wt% 5TCzBN: 1 wt% 2TCzBN/T2T/NBphen/CsF/Al | 4.2 | 23.1 | LT50 = 456.3(1,000 nit) | (0.14, 0.34) | [123] |
ITO/PEDOT: PSS/FPCz-V/MADN: 2 wt% M-t-DABNA/ANT-BIZ/Liq/Al | 2.3 | 7.0 | LT95 = 153(1,000 nit) | (0.13, 0.09) | [124] |
ITO/PEDOT: PSS/LBH001: 3 wt% m-DABNA-AdCz/TPBi/Yb/Ag | 2.8 | - | LT95 = 55.7(1,000 nit) | (0.133, 0.076) | [125] |
EL: electroluminescence; OLEDs: organic light-emitting diodes; EQE: external quantum efficiency; CIE: Commission Internationale de l'Eclairage.
Device structure | Von (V) | EQEmax (%) | Lifetime (h) | CIE (x,y) | Ref. |
ITO/HAT-CN/CPD/mCBP: 18 Vol%-14 Vol% Ir(dmp)3/mCBP: 11 Vol%-9 Vol% Ir(pmp)3: 3 Vol% mer-Ir(pmp)3/mCBP: 12 Vol%-8 Vol% Ir(dmp)3/mCBP: 8 Vol% Ir(dmp)3/mCBP/Alq3/Liq/Al | - | 9.6 | LT80 = 334(1,000 nit) | (0.16, 0.30) | [40] |
ITO/HAT-CN/mCBP: 18 Vol%-8 Vol% Ir(dmp)3/mCBP/Alq3/Alq3: 2 Vol% Li/HAT-CN/mCBP: 18 Vol%-8 Vol% Ir(dmp)3/mCBP/Alq3/Liq/Al | - | 18 | LT80 = 616(1,000 nit) | (0.15, 0.29) | [44] |
ITO/PPBI/NPD/4DBFHPB/mCBP: 30 wt% 5TCzBN/DBT-TRZ/DBP: 20 wt% Liq/Libpp/Al | 2.74 | 21.5 | LT50 = 1,700(500 nit) | - | [53] |
ITO/HAT-CN/α-NPD/Tris-PCz/mCB/SF3-TRZ: 30 wt% BCz-TRZ/SF3-TRZ/Bebq2/LiF/Al | 2.62 | 8.8 | LT50 = 454(1,000 nit) | (0.20, 0.36) | [61] |
ITO/TAPC/TCTA/mCP/DPEPO: 15 wt% p4TCzPhBN/DPEPO/Bphen/LiF/Al | - | 22.8 | LT80 = 40.4(500 nit) | (0.15, 0.19) | [79] |
ITO/HATCN/NPB/TCTA/mCPCz: 40 wt% p4TCzPhBN: 2 wt% t-DABNA/CzPhPy/DPPyA: Liq (1:1)/LiF/Al | - | 32.5 | LT80 = 60(1,000 nit) | (0.13, 0.12) | [79] |
ITO/HATCN/TAPC/Tris-PCz/mCBP/mCBP: 20 wt% HDT-1: 0.5 wt% v-DABNA/SF3-TRZ/SF3-TRZ: Liq/Liq/Al/HATCN/TAPC/Tris-Pcz/mCBP/mCBP: 20 wt% HDT-1: 0.5 wt% v-DABNA/SF3-TRZ/SF3TRZ: Liq/Liq/Al | 6.5 | 41.0 | LT95 = 18(1,000 nit) | (0.13, 0.16) | [80] |
ITO/HATCN/BCFN/SiCzCz/SiCzCz: SiTrzCz2: D-5CzBN (55:30:15)/mSiTRZ/DPPyA: Liq/LiF/Al | - | 29.0 | LT80 = 210(1,000 nit) | (0.16, 0.24) | [81] |
ITO/HATCN/BCFN/SiCzCz/SiCzCz: SiTrzCz2: D-5tCzBN (55:30:15)/mSiTRZ/DPPyA: Liq/LiF/Al | - | 29.2 | LT80 = 949(1,000 nit) | (0.17, 0.28) | [81] |
ITO/HATCN/BCFN/SiCzCz/SiCzCz: SiTrzCz2: 4CzBN-PhCN (48:32:20)/SiTrzCz2/DPPyA: Liq/LiF/Al | - | 28.0 | LT95 = 26.7(5,000 nit) | (0.21, 0.40) | [82] |
ITO/HATCN/BCFN/SiCzCz/SiCzCz: SiTrzCz2: 4tCzBN-PhCN (48:32:20)/SiTrzCz2/DPPyA: Liq/LiF/Al | - | 37.1 | LT95 = 37.6(5,000 nit) | (0.23, 0.48) | [82] |
ITO/HATCN/BCFN/SiCzCz/SiCzCz: SiTrzCz2: 4tCzBN-TPTRZ (48:32:20)/SiTrzCz2/DPPyA: Liq/LiF/Al | - | 27.9 | LT95 = 16.4(5,000 nit) | (0.23, 0.44) | [82] |
ITO/DNTPD/BPBPA/mCBP/oCBP: CNmCBPCN: PCz-Trz: v-DABNA (50:50:10:1)/DBFTrz/ZADN/LiF/Al | - | 33.5 | LT50 = 113(1,000 nit) | (0.12, 0.18) | [83] |
ITO/DNTPD/BPBPA/mCBP/oCBP: CNmCBPCN: PPCz-Trz: v-DABNA (50:50:10:1)/DBFTrz/ZADN/LiF/Al | - | 33.0 | LT50 = 151(1,000 nit) | (0.13, 0.20) | [83] |
ITO/HAT-CN/BCFN/SiCzCz/SiTrzCz2: SiCzCz: BD-02 (0.60:0.27:0.13)/mSiTrz/mSiTrz: Liq (5:5)/LiF/Al | 2.8 | 25.4 | LT70 = 1,113(1,000 nit) | (0.141, 0.197) | [106] |
ITO/HATCN/BCFN/SiCzCz/SiCzCz: SiTrzCz2: PtON-TBBI: TBE01 (65:35:13:0.4)/2SiTrzPh/2SiTrzPh: Liq(5:5)/LiF/Al | - | 25.4 | LT95 = 42.3(1,000 nit) | (-, 0.165) | [107] |
ITO/HATCN/BCFN/SiCzCz/SiCzCz: SiTrzCz2: PtON-TBBI: TBE02 (65:35:13:0.4)/2SiTrzPh/2SiTrzPh: Liq(5:5)/LiF/Al | - | 25.8 | LT95 = 72.9(1,000 nit) | (-, 0.165) | [107] |
ITO/HATCN/Tris-PCz/mCBP/mCBP: 20 wt% 3Cz2DPhCzBN/SF3-TRZ/SF3-TRZ: Liq/Liq/Al | - | 20.9 | LT97 = 110(1,000 nit) | (0.21, 0.44) | [129] |
ITO/HATCN/Tris-PCz/mCBP/mCBP: 20 wt% 3Ph2CzCzBN/SF3-TRZ/SF3-TRZ: 30 wt% Liq/Liq/Al | 4.0 | 17.8 | LT90 = 38(1,000 nit) | (0.17, 0.36) | [130] |
ITO/HAT-CN/α-NPD/Tris-PCz/mCBP/mCBP: 15 wt% 5CzTRZ/CF3-TRZ/BPPB: 30 wt% Liq/Liq/Al | - | 29.3 | LT90 = 35.4(5,000 nit) | - | [131] |
EL: electroluminescence; OLEDs: organic light-emitting diodes; EQE: external quantum efficiency; CIE: Commission Internationale de l'Eclairage.
Efficiency and lifetime are critical performance parameters of blue OLEDs, and they are inherently linked to the behavior of charge and excitons. Although blue OLEDs based on different types of emitters exhibit distinct performance advantages, simultaneously achieving high-performance and long lifetime blue OLEDs still confront various challenges. Regulating the behavior of charge and exciton appropriately has demonstrated a great potential to enhance the efficiency and lifetime. Notably, the need for balanced charge transport and high exciton utilization remains consistent across all high-performance blue OLEDs. Given that the host material significantly influences charge transport and exciton dynamics within the EML, the host engineering for high-performance blue OLEDs has been thoroughly explored. The charge and exciton management strategies for high-performance, long-lifetime blue OLEDs are summarized in Figure 21. Although some progress has been made in the development of blue OLEDs, future-oriented charge and exciton management strategies are still required to facilitate the realization of high-efficiency and long-lifetime blue OLEDs at a commercial level.
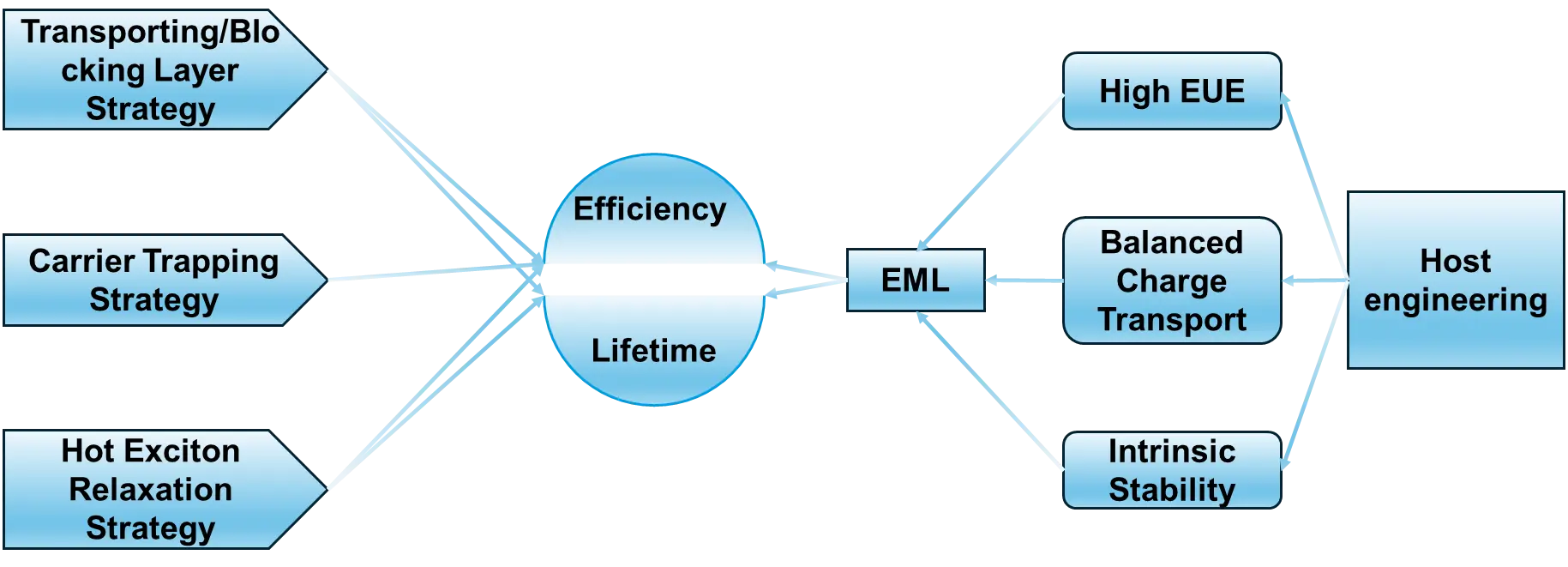
Figure 21. Schematic illustration of charge and exciton management strategies for high-performance, long-lifetime blue OLEDs. OLEDs: organic light-emitting diodes; EML: emission layer; EUE: exciton utilization efficiency.
First, the emission of devices should primarily occur via energy transfer rather than direct carrier trapping by the emitter. Generally, with appropriate device design, devices dominated by either of these emission mechanisms can achieve comparable exciton utilization efficiency. However, as mentioned earlier, carrier trapping negatively impacts device lifetime. An effective strategy is to use an exciplex with a high triplet energy as the host for blue OLEDs and to minimize the HOMO/LUMO level difference between the exciplex host and emitter. This approach facilitates emission dominated by energy transfer while reducing carrier capture on the emitter in the EML. Additionally, electroplexes, as well as assistant hosts, can also serve as primary exciton generation sites, leading to efficient emission through energy transfer to the emitter.
Second, the triplet excitons must be rapidly harvested to produce the emission of light. A direct approach is to improve the triplet exciton utilization of the blue emitter, such as by accelerating the RISC process in hot exciton materials and TADF materials. Additionally, introducing TADF assistant hosts and phosphorescent assistant hosts into the EML to increase the number of energy transfer pathways is an effective strategy to accelerate triplet exciton utilization.
Moreover, the energy transfer processes within the EML and between the EML and adjacent layers must be precisely regulated to minimize the formation of non-radiative triplet excitons and long-lived triplet excitons. For example, by employing device designs with spatial separation or EML materials containing bulky substituent groups to appropriately increase the intermolecular distance between the host and emitter, it is possible to maintain efficient FRET process while suppressing the DET process, which enhances the performance of singlet-radiative blue OLEDs.
Third, the exciton recombination zone should be stably confined within the EML and should be relatively broad. Designing hosts and transport layer materials with high triplet energy levels, strong BDE, and excellent charge transport properties is crucial to achieving this goal. Notably, the stability of the blue emitter also affects the long-term charge transport balance within the EML. Consequently, stable and high triplet energy levers exciplex as hosts, the component of exciplex as the transport layers, are widely applied in high-performance blue OLEDs. Additionally, the development of stable blue MRTADF emitters should be pursued to facilitate the construction of a stable EML.
In summary, high-performance and long-lifetime blue OLEDs are expected to comprise a stable deep-blue exciplex host, a blue TADF material or deep-blue phosphorescent material is served as the assistant host, combining with a blue MR-TADF material with high radiative transition rate as the terminal emitter, which facilitate the rapid utilization of triplet excitons. Notably, introducing stable TADF materials as components of the exciplex can also enhance the performance of blue devices. Moreover, the n-type and p-type hosts of the exciplexes, can be used as transport or blocking layers, promoting balanced charge transport and preventing exciton leakage. Additionally, designing exciton management strategies to promote FRET and suppress DET processes within the EML can further enhance the efficiency and lifetime performance of blue OLEDs. With the development of universally applicable charge and exciton management strategies and the optimization of host engineering, blue OLEDs that overcome efficiency and lifespan limitations will enable broader commercialization of OLED technology.
Authors contribution
Li Z: Draft Writing.
Liu D: Discussion and optimization of the manuscript.
Su S: Manuscript review and revision.
Conflicts of interest
The authors declare no conflicts of interest.
Ethical approval
Not applicable.
Consent to participate
Not applicable.
Consent for publication
Not applicable.
Availability of data and materials
Not applicable.
Funding
The authors greatly appreciate the financial support from the National Key R & D Program of China (2020YFA0714600), the National Natural Science Foundation of China (52273179, 52303228, U23A20594, and 51625301), and the China National Postdoctoral Program for Innovative Talents (BX20230129).
Copyright
© The Author(s) 2024.
References
-
1. Wang Y, Yun JH, Wang L, Lee JY. High triplet energy hosts for blue organic light-emitting diodes. Adv Funct Mater. 2021;31(12):2008332.
[DOI] -
2. Siddiqui I, Kumar S, Tsai YF, Gautam P, Shahnawaz , Kesavan K, et al. Status and challenges of blue OLEDs: a review. Nanomaterials. 2023;13(18):2521.
[DOI] -
3. Hu YN, Fan XC, Huang F, Shi YZ, Wang H, Cheng YC, et al. Novel multiple resonance type TADF emitter as blue component for highly efficient blue-hazard-free white organic light-emitting diodes. Adv Opt Mater. 2023;11(3):2202267.
[DOI] -
4. Im Y, Byun SY, Kim JH, Lee DR, Oh CS, Yook KS, et al. Recent progress in high-efficiency blue-light-emitting materials for organic light-emitting diodes. Adv Funct Mater. 2017;27(13):1603007.
[DOI] -
5. Tang CW, Vanslyke SA. Organic electroluminescent diodes. Appl Phys Lett. 1987;51(12):913-915.
[DOI] -
6. Giebink NC, D'Andrade BW, Weaver MS, Mackenzie PB, Brown JJ, Thompson ME, et al. Intrinsic luminance loss in phosphorescent small-molecule organic light emitting devices due to bimolecular annihilation reactions. J Appl Phys. 2008;103(4):044509.
[DOI] -
7. Giebink NC, D'Andrade BW, Weaver MS, Brown JJ, Forrest SR. Direct evidence for degradation of polaron excited states in organic light emitting diodes. J Appl Phys. 2009;105(12):124514.
[DOI] -
8. Jacquemin D, Escudero D. The short device lifetimes of blue PhOLEDs: insights into the photostability of blue Ir(iii) complexes. Chem Sci. 2017;8(11):7844-7850.
[DOI] -
9. Baldo MA, Adachi C, Forrest SR. Transient analysis of organic electrophosphorescence. II. Transient analysis of triplet-triplet annihilation. Phys Rev B. 2000;62(16):10967.
[DOI] -
10. Jiang J, Lee JY. Degradation mechanisms and lifetime extending strategy of phosphorescent and thermally activated delayed-fluorescence organic light-emitting diodes. Mater Today. 2023;68:204-233.
[DOI] -
11. Song W, Lee JY. Degradation mechanism and lifetime improvement strategy for blue phosphorescent organic light-emitting diodes. Adv Opt Mater. 2017;5(9):1600901.
[DOI] -
12. Hwang KM, Kim T, Kang S. A systematic investigation to unravel the primary determinant of the operational stability of blue fluorescent organic light-emitting diodes. J Mater Chem C. 2022;10(27):10139-10146.
[DOI] -
13. Song YN, Kang S, Kim T. Exceeding 10000 h of lifetime in blue fluorescent organic light-emitting diodes by introducing an electron leakage pathway. Adv Opt Mater. 2023;11(19):2301193.
[DOI] -
14. Lee JH, Chen CH, Lee PH, Lin HY, Leung MK, Chiu TL, et al. Blue organic light-emitting diodes: current status, challenges, and future outlook. J Mater Chem C. 2019;7(20):5874-5888.
[DOI] -
15. Swayamprabha SS, Dubey DK, Shahnawaz , Yadav RAK, Nagar MR, Sharma A, et al. Approaches for long lifetime organic light emitting diodes. Adv Sci. 2021;8(1):2002254.
[DOI] -
16. Kondakov DY. Triplet-triplet annihilation in highly efficient fluorescent organic light-emitting diodes: current state and future outlook. Philos Trans R Soc A. 2015;373(2044):20140321.
-
17. Bai K, Li M, Tan X, Dai L, Liang K, Li H, et al. Reducing intersystem crossing rates of boron emitters for high-efficiency and long-lifetime deep-blue OLEDs. J Mater Chem C. 2023;11(46):16159-16167.
[DOI] -
18. Baldo MA, Thompson ME, Forrest SR. High-efficiency fluorescent organic light-emitting devices using a phosphorescent sensitizer. Nature. 2000;403(6771):750-753.
[DOI] -
19. Nakanotani H, Higuchi T, Furukawa T, Masui K, Morimoto K, Numata M, et al. High-efficiency organic light-emitting diodes with fluorescent emitters. Nat Commun. 2014;5:4016.
[DOI] -
20. Zhang D, Duan L, Li C, Li Y, Li H, Zhang D, et al. High-efficiency fluorescent organic light-emitting devices using sensitizing hosts with a small singlet-triplet exchange energy. Adv Mater. 2014;26(29):5050-5055.
[DOI] -
21. Nalaoh P, Sungworawongpana N, Chasing P, Waengdongbung W, Funchien P, Kaiyasuan C, et al. A dimeric π-stacking of anthracene inducing efficiency enhancement in solid-state fluorescence and non-doped deep-blue triplet-triplet annihilation organic light-emitting diodes. Adv Opt Mater. 2021;9(17):2100500.
[DOI] -
22. Xing L, Zhu ZL, He J, Qiu Z, Yang Z, Lin D, et al. Anthracene-based fluorescent emitters toward superior-efficiency nondoped TTA-OLEDs with deep blue emission and low efficiency roll-off. Chem Eng J. 2021;421(2):127748.
[DOI] -
23. Yang GX, Liu DH, Gu Q, Peng X, Li DL, Li M, et al. TripletTriplet annihilation enhanced deep-blue organic light-emitting diodes by naphtho[1,2-d]imidazole-isomer derivatives with spin-orbit coupling. Adv Opt Mater. 2023;11(18):2300455.
[DOI] -
24. Chen CH, Li YS, Fang SC, Lin BY, Li CY, Liao YC, et al. High-Performance deep-blue OLEDs harnessing triplet-triplet annihilation under low dopant concentration. Adv Photonics Res. 2023;4(2):2200204.
[DOI] -
25. Chen CH, Hsiao ZC, Fan BA, Hou LJ, Lin JH, Lin BY, et al. Benzimidazole-substituted bisanthracene: a highly efficient deep-blue triplet-triplet fusion OLED emitter at low dopant concentration. Mater Today Chem. 2022;26:101185.
[DOI] -
26. Nguyen TB, Nakanotani H, Adachi C. An overlooked charge-transfer interaction in the interfacial triplet-triplet upconversion process in blue organic light-emitting diodes. Adv Opt Mater. 2022;10(18):2200704.
[DOI] -
27. Sato T, Miyamae T, Ohata H, Tsutsui T. Direct observations of the charge behavior of a high-efficiency blue organic light-emitting diode under operating conditions using electric-field-induced doubly resonant sum-frequency-generation vibrational spectroscopy. Org Electron. 2019;74:118-125.
[DOI] -
28. Wu Y, Xiao S, Guo K, Qiao X, Yang D, Dai Y, et al. Understanding the degradation mechanism of TTA-based blue fluorescent OLEDs by exciton dynamics and transient electroluminescence measurements. Phys Chem Chem Phys. 2023;25(43):29451-29458.
[DOI] -
29. Wu G, Ge X, Yang Z, Liu Y, Chen Z, Wang Y, et al. High-efficiency blue hybridized local and charge-transfer fluorescent material affording OLEDs with external quantum efficiency exceeding 14 %. Chem Eng J. 2024;497:154659.
[DOI] -
30. Zhang L, Wang L, Samedov K, Chen M, Chen D, Cai Y. High-Lying triplet excitons utilization of silole derivatives enables their efficiency breakthrough in OLEDs. Adv Funct Mater. 2024;34(51):2410250.
[DOI] -
31. Liao C, Chen B, Xie Q, Li X, Liu H, Wang S. A breakthrough in solution-processed ultra-deep-blue HLCT OLEDs: A record external quantum efficiency exceeding 10% based on novel v-shaped emitters. Adv Mater. 2023;35(48):2305310.
[DOI] -
32. Lv J, Song S, Li J, Peng L, Li Y, Liu Y, et al. High and balanced bipolar-transporting deep-blue HLCT material for efficient monochrome and white OLEDs based on a simple phenanthroimidazole-dibenzothiophene derivative. Adv Opt Mater. 2024;12(2):2301413.
[DOI] -
33. Xu Y, Xu P, Hu D, Ma Y. Recent progress in hot exciton materials for organic light-emitting diodes. Chem Soc Rev. 2021;50(2):1030-1069.
[DOI] -
34. Yu Y, Xu P, Pan Y, Qiao X, Ying L, Hu D, et al. Pyrene-based emitters with ultrafast upper-level triplet-singlet intersystem crossing for high-efficiency, low roll-off blue organic light-emitting diode. Adv Opt Mater. 2023;11(4):2202217.
[DOI] -
35. Du C, Liu H, Cheng Z, Zhang S, Qu Z, Yang D, et al. Ultraefficient non-doped deep blue fluorescent OLED: Achieving a high EQE of 10.17% at 1000 cd m-2 with CIEy < 0.08. Adv Funct Mater. 2023;33(45):2304854.
[DOI] -
36. Xu L, Yu Y, Li M, Li Y, Tan W, Wang B, et al. Pyrene-Based emitter with a small energy gap between high-energy triplet and singlet state for high-performance blue organic light-emitting diodes. Adv Opt Mater. 2024;12(29):2401275.
[DOI] -
37. Li T, Ravinson DSM, Haiges R, Djurovich PI, Thompson ME. Enhancement of the luminescent efficiency in carbene-Au(I)-aryl complexes by the restriction of renner-teller distortion and bond rotation. J Am Chem Soc. 2020;142(13):6158-6172.
[DOI] -
38. Calupitan JP, Poirot A, Wang J, Delavaux-Nicot B, Wolff M, Jaworska M, et al. Mechanical modulation of the solid-state luminescence of tricarbonyl Rhenium(I) complexes through the interplay between two triplet excited states. Chem Eur J. 2021;27(12):4191-4196.
[DOI] -
39. Cao L, Klimes K, Ji Y, Fleethan T, Li J. Efficient and stable organic light-emitting devices employing phosphorescent molecular aggregates. Nat Photonics. 2021;15:230-237.
[DOI] -
40. Lee J, Jeong C, Batagoda T, Coburn C, Thompson ME, Forrest SR. Hot excited state management for long-lived blue phosphorescent organic light-emitting diodes. Nat Commun. 2017;8:15566.
[DOI] -
41. Wu C, Shi K, Li S, Yan J, Feng ZQ, Tong KN, et al. Design strategies of iridium(III) complexes for highly efficient saturated blue phosphorescent OLEDs with improved lifetime. EnergyChem. 2024;6(2):100120.
[DOI] -
42. Seo JA, Jeon SK, Gong MS, Lee JY, Noh CH, Kim SH. Long lifetime blue phosphorescent organic light-emitting diodes with an exciton blocking layer. J Mater Chem C. 2015;3(18):4640-4645.
[DOI] -
43. Choi JM, Lee JY. Triplet emitter doped exciton harvesting layer for improved efficiency and long lifetime in blue phosphorescent organic light-emitting diodes. Synth Met. 2016;220:573-577.
[DOI] -
44. Zhang Y, Lee J, Forrest SR. Tenfold increase in the lifetime of blue phosphorescent organic light-emitting diodes. Nat Commun. 2014;5:5008.
[DOI] -
45. Klimes K, Zhu ZQ, Li J. Efficient blue phosphorescent OLEDs with improved stability and color purity through judicious triplet exciton management. Adv Funct Mater. 2019;29(31):1903068.
[DOI] -
46. Han SH, Lee KH, Lee JY. Spatial separation of two blue triplet emitters for improved lifetime in blue phosphorescent organic light-emitting diodes by confining excitons at the interface between two emitting layers. Org Electron. 2019;69:227-231.
[DOI] -
47. Zhang Y, Aziz H. Degradation mechanisms in blue phosphorescent organic light-emitting devices by exciton-polaron interactions: loss in quantum yield versus loss in charge balance. ACS Appl Mater Interfaces. 2017;9(1):636-643.
[DOI] -
48. Song W, Lee JY. Lifetime extension of blue phosphorescent organic light-emitting diodes by suppressing triplet-polaron annihilation using a triplet emitter doped hole transport layer. Org Electron. 2017;49:152-156.
[DOI] -
49. Song W, Kim T, Lee Y, Lee J. Degradation mechanism study and electron scattering device structure for long lifetime in blue phosphorescent organic light-emitting diodes. Org Electron. 2017;43:82-86.
[DOI] -
50. Hatakeyama T, Shiren K, Nakajima K, Nomura S, Nakatsuka S, Kinoshita K, et al. Ultrapure blue thermally activated delayed fluorescence molecules: efficient HOMO-LUMO separation by the multiple resonance effect. Adv Mater. 2016;28(14):2777-2781.
[DOI] -
51. Ochi J, Yamasaki Y, Tanaka K, Kondo Y, Isayama K, Oda S, et al. Highly efficient multi-resonance thermally activated delayed fluorescence material toward a BT.2020 deep-blue emitter. Nat Commun. 2024;15:2361.
[DOI] -
52. Kondo Y, Yoshiura K, Kitera S, Nishi H, Oda S, Gotoh H, et al. Narrowband deep-blue organic light-emitting diode featuring an organoboron-based emitter. Nat Photonics. 2019;13:678-682.
[DOI] -
53. Kamata T, Sasabe H, Ito N, Sukegawa Y, Arai A, Chiba T, et al. Simultaneous realization of high-efficiency, low-drive voltage, and long lifetime TADF OLEDs by multifunctional hole-transporters. J Mater Chem C. 2020;8(21):7200-7210.
[DOI] -
54. Jiang Z, Yan H, Zhang X, Meng Z, Kuang C, Zhang X, et al. Novel spiro core-based hole transport materials for stable deep-blue OLEDs with LT95 over 420 h. Adv Opt Mater. 2023;11(23):2301014.
[DOI] -
55. Tanaka M, Noda H, Nakanotani H, Adachi C. Effect of carrier balance on device degradation of organic light-emitting diodes based on thermally activated delayed fluorescence emitters. Adv Electron Mater. 2019;5(5):1800708.
[DOI] -
56. Chung WJ, Lee JY. Highly efficient and stable blue organic light-emitting diodes through the selective quenching of long-living triplet exciton of a thermally activated delayed fluorescence emitter. J Mater Chem C. 2021;9(23):7458-7464.
[DOI] -
57. Lim J, Lee KH, Kim JM, Lee JY. Synergistic control of hot exciton relaxation and exciton-polaron dispersion using a polaron retarder for long lifetime in thermally activated delayed fluorescence organic light-emitting diodes. Adv Opt Mater. 2022;10(22):2201575.
[DOI] -
58. Liu Z, Cao F, Tsuboi T, Yue Y, Deng C, Ni X, et al. A high fluorescence rate is key for stable blue organic light-emitting diodes. J Mater Chem C. 2018;6(29):7728-7733.
[DOI] -
59. Li YZ, Liang HC, Chen CH, Chiu CH, Huang LC, Lee YT, et al. High efficiency in blue TADF OLED using favorable horizontal oriented host. Chem Eng J. 2024;498(15):155553.
[DOI] -
60. Nakanotani H, Masui K, Nishide J, Shibata T, Adachi C. Promising operational stability of high-efficiency organic light-emitting diodes based on thermally activated delayed fluorescence. Sci Rep. 2013;3:2127.
[DOI] -
61. Cui LS, Ruan SB, Bencheikh F, Nagata R, Zhang L, Inada K, et al. Long-lived efficient delayed fluorescence organic light-emitting diodes using n-type hosts. Nat Commun. 2017;8:2250.
[DOI] -
62. Byeon SY, Han SH, Lee JY. Negative polaron-stabilizing host for improved operational lifetime in blue phosphorescent organic light-emitting diodes. Adv Opt Mater. 2017;5(20):1700387.
[DOI] -
63. Kim DS, Lee KH, Lee JY. Novel positive polaron stabilizing n-type host for high efficiency and long lifetime in blue phosphorescent organic light-emitting diodes. ACS Appl Mater Interfaces. 2020;12(17):19737-19745.
[DOI] -
64. Lee H, Park JH, Yang KJ, Hwang SJ, Braveenth R, Ha TH, et al. CN-substituted ortho-terphenyl core based high triplet energy bipolar host materials for stable and efficient blue TADF devices. J Mater Chem C. 2021;9(23):7426-7435.
[DOI] -
65. Kuang C, Li S, Murtaza I, Meng Z, Li H, Zhang X, et al. Enhanced horizontal dipole orientation by novel penta-helicene anthracene-based host for efficient blue fluorescent OLEDs. Small. 2024;20(24):2311114.
[DOI] -
66. Bezvikonnyi O, Bucinskas A, Arsenyan P, Petrenko A, Wei ZY, Lee JH, et al. Enhancement of blue doping-free and hyperfluorescent organic light emitting diode performance through triplet-triplet annihilation in the derivatives of anthracene and carbazole. ACS Appl Electron Mater. 2024;6(6):4489-4503.
[DOI] -
67. Cai W, Li W, Song X, Zheng X, Guo H, Lin C, et al. Host engineering of deep-blue-fluorescent organic light-emitting diodes with high operational stability and narrowband emission. Adv Sci. 2024;11(43):2407278.
[DOI] -
68. Guo K, Lin C, Wu Y, Xiao S, Qiao X, Yang D, et al. Understanding of degradation mechanism by exciton dynamics and enhancement of operational lifetime by exciton management in blue fluorescent OLEDs based on hybridized local and charge-transfer molecule. Adv Opt Mater. 2023;11(8):2202988.
[DOI] -
69. Lv J, Li J, Wang S, Shen H, Xia L, Xue S, et al. An efficient and stable deep-blue oxygen-bridged triphenylborane-based fluorophore with hybridized local and charge-transfer states. J Mater Chem C. 2024;12(43):17475-17481.
[DOI] -
70. Lim H, Woo SJ, Ha YH, Kim YH, Kim JJ. Breaking the efficiency limit of deep-blue fluorescent OLEDs based on anthracene derivatives. Adv Mater. 2022;34(1):2100161.
[DOI] -
71. Xie D, Wang Z, Chen Y, Qiao X, Yang D, Sun Q, et al. High efficiency triplet-triplet fusion blue fluorescence OLEDs by introducing a "Hot Exciton" efficiency enhancement layer. Adv Opt Mater. 2024;12(21):2400561.
[DOI] -
72. Nguyen TB, Nakanotani H, Chan CY, Kakumachi S, Adachi C. Enhancing triplet-triplet upconversion efficiency and operational lifetime in blue organic light-emitting diodes by utilizing thermally activated delayed fluorescence materials. ACS Appl Mater Interfaces. 2023;15(19):23557-23563.
[DOI] -
73. Kang S, Lee JY, Kim T. Unveiling the root cause of the efficiency-lifetime trade-off in blue fluorescent organic light-emitting diodes. Electron Mater Lett. 2020;16:1-8.
[DOI] -
74. Tasaki S, Nishimura K, Toyoshima H, Masuda T, Nakamura M, Nakano Y, et al. Realization of ultra-high-efficient fluorescent blue OLED. J Soc Inf Disp. 2022;30(5):441-451.
[DOI] -
75. Lee SH, Chae MY, Jung YH, Oh JH, Kim HR, Naveen KR, et al. Enhanced triplet-triplet fusion for high efficiency and long lifetime of multiresonant pure blue organic light emitting diodes. J Ind Eng Chem. 2023;122:452-458.
[DOI] -
76. Li B, Lou J, Zhang H, Li G, He X, Huang Y, et al. "Exciton recovery" strategy in hot exciton emitter toward high-performance non-doped deep-blue and host-sensitized organic light-emitting diodes. Adv Funct Mater. 2023;33(12):2212876.
[DOI] -
77. Yang J, Peng X, Liu D, Chen J, Qiu W, Yang X, et al. Realizing stable narrowband emission blue fluorescent organic light-emitting diodes with dual-channel triplet exciton harvesting host. J Phys Chem C. 2024;128(12):5330-5337.
[DOI] -
78. Zhang D, Song X, Cai M, Kaij H, Duan L. Versatile indolocarbazole-isomer derivatives as highly emissive emitters and ideal hosts for thermally activated delayed fluorescent OLEDs with alleviated efficiency roll-off. Adv Mater. 2018;30(7):1705406.
[DOI] -
79. Zhang D, Song X, Gillett AJ, Drummond BH, Jones STE, Li G, et al. Efficient and stable deep-blue fluorescent organic light-emitting diodes employing a sensitizer with fast triplet upconversion. Adv Mater. 2020;32(19):1908355.
[DOI] -
80. Chan CY, Tanaka M, Lee YT, Wong YW, Nakanotani H, Hatakeyama T, et al. Stable pure-blue hyperfluorescence organic light-emitting diodes with high-efficiency and narrow emission. Nat Photonics. 2021;15:203-207.
[DOI] -
81. Huang T, Wang Q, Zhang H, Zhang Y, Zhan G, Zhang D, et al. Enhancing the efficiency and stability of blue thermally activated delayed fluorescence emitters by perdeuteration. Nat Photonics. 2024;18:516-523.
[DOI] -
82. Huang T, Wang Q, Zhang H, Xin Y, Zhang Y, Chen X, et al. Delocalizing electron distribution in thermally activated delayed fluorophors for high-efficiency and long-lifetime blue electroluminescence. Nat Mater. 2024;23:1523-1530.
[DOI] -
83. Jeon SO, Lee KH, Kim JS, Ihn SG, Chung YS, Kim JW, et al. High-efficiency, long-lifetime deep-blue organic light-emitting diodes. Nat Photonics. 2021;15:208-215.
[DOI] -
84. Lee YT, Chan CY, Tanaka M, Mamada M, Goushi K, Tang X, et al. Tailor-made multi-resonance terminal emitters toward narrowband, high-efficiency, and stable hyperfluorescence organic light-emitting diodes. Adv Opt Mater. 2022;10(17):2200682.
[DOI] -
85. Zuo P, Qu YK, Zheng Q, Liao LS, Jiang ZQ. Sensitized organic light-emitting diodes: towards high efficiency and long lifetimes. Mater Chem Front. 2023;7(9):1760-1780.
[DOI] -
86. Ahn DH, Jeong JH, Song J, Lee JY, Kwon JH. Highly efficient deep blue fluorescent organic light-emitting diodes boosted by thermally activated delayed fluorescence sensitization. ACS Appl Mater Interfaces. 2018;10(12):10246-10253.
[DOI] -
87. Heimel P, Mondal A, May F, Kowalsky W, Lennartz C, Andrienko D, et al. Unicolored phosphor-sensitized fluorescence for efficient and stable blue OLEDs. Nat Commun. 2018;9:4990.
[DOI] -
88. Kim KJ, Lee H, Kang S, Kim T. Superbly long lifetime over 13,000 h for multiple energy transfer channels in deep blue phosphorescence organic light-emitting diodes with Ir complex under CIEy of 0.17. Chem Eng J. 2022;448:137671.
[DOI] -
89. Nam S, Kim JW, Bae HJ, Maruyama MY, Jeong D, Kim JH, et al. Improved efficiency and lifetime of deep-blue hyperfluorescent organic light-emitting diode using Pt(II) complex as phosphorescent sensitizer. Adv Sci. 2021;8(16):2100586.
[DOI] -
90. Lee KH, Lee JY. Phosphor sensitized thermally activated delayed fluorescence organic light-emitting diodes with ideal deep blue device performances. J Mater Chem C. 2019;7(28):8562-8568.
[DOI] -
91. You C, Wang XQ, Zhou X, Yuan Y, Liao LS, Liao YC, et al. Homoleptic Ir(III) phosphors with 2-phenyl-1,2,4-triazol-3-ylidene chelates for efficient blue organic light-emitting diodes. ACS Appl Mater Interfaces. 2021;13(49):59023-59034.
[DOI] -
92. Chung WJ, Lee KH, Jung M, Lee KM, Park HC, Eum MS, et al. Over 30 000 h device lifetime in deep blue organic light-emitting diodes with y color coordinate of 0.086 and current efficiency of 37.0 cd A-1. Adv Opt Mater. 2021;9(13):2100203.
[DOI] -
93. Qin Y, Yang X, Jin J, Li D, Zhou X, Zheng Z, et al. Facially coordinated, tris-bidentate purin-8-ylidene Ir(III) complexes for blue electrophosphorescence and hyperluminescence. Adv Opt Mater. 2022;10(23):2201633.
[DOI] -
94. Baek SH, Park JY, Woo SJ, Lee WS, Kim WS, Cheon HJ, et al. Synergistic enhancement of emitting dipole orientation between Pt-based phosphorescent sensitizers and boron-based multi-resonance fluorescent emitters for high-performance phosphor-sensitized fluorescent organic light-emitting diodes. Small Struct. 2024;5(6):2300564.
[DOI] -
95. Yang K, Nam S, Kim J, Kwon ES, Jung Y, Choi H, et al. Effects of charge dynamics in the emission layer on the operational lifetimes of blue phosphorescent organic light-emitting diodes. Adv Funct Mater. 2022;32(19):2108595.
[DOI] -
96. Ko D, Lee J. Trap-dependent electrical characteristics of organic semiconductor devices. SID Symp Dig Tech Pap. 2020;51(1):2121-2124.
[DOI] -
97. Sohn JH, Ko D, Lee H, Han J, Lee SD, Lee C. Degradation mechanism of blue thermally activated delayed fluorescent organic light-emitting diodes under electrical stress. Org Electron. 2019;70:286-291.
[DOI] -
98. Zee B, Li Y, Wetzelaer GJAH, Blom PWM. Triplet-polaron-annihilation-Induced degradation of organic light-emitting diodes based on thermally activated delayed fluorescence. Phys Rev Appl. 2022;18(6):064002.
[DOI] -
99. Lu X, Wang Q, Cai X, Qu Y, Li Z, Li C, et al. Exciplex hosts for constructing green multiple resonance delayed fluorescence OLEDs with high color purity and low efficiency roll-offs. Adv Funct Mater. 2024;34(18):2313897.
[DOI] -
100. Liu W, Pan J, Sun Q, Dai Y, Yang D, Qiao X, et al. Exciton dynamics of the efficiency roll-off of exciplex-based OLEDs and low efficiency roll-off phosphorescence OLEDs based on an exciplex as the host. J Mater Chem C. 2024;12(32):12317-12324.
[DOI] -
101. Sarma M, Chen LM, Cheng YS, Wong KT. Exciplexes in OLEDs: Principles and promises. Mater Sci Eng R Rep. 2022;150:100689.
[DOI] -
102. Zhang M, Zheng CJ, Lin H, Tao SL. Thermally activated delayed fluorescence exciplex emitters for high-performance organic light-emitting diodes. Mater Horiz. 2021;8(2):401-425.
[DOI] -
103. Choi KH, Lee KH, Lee JY, Kim TY. Simultaneous achievement of high efficiency and long lifetime in deep blue phosphorescent organic light-emitting diodes. Adv Opt Mater. 2019;7(23):1901374.
[DOI] -
104. Yun JH, Lee KH, Chung WJ, Lee JY, Lee Y, Lyu JJ. Thermally activated delayed fluorescence type exciplex host for long lifetime in deep blue phosphorescent organic light-emitting diodes. Chem Eng J. 2021;417:128086.
[DOI] -
105. Kim DJ, Baek SH, Kim WS, Ahn KH, Lee JH. Bicarbazole-triazine hybrid type mixed host materials for blue phosphorescent OLEDs with enhanced efficiency and lifetime. J Mater Chem C. 2022;10(22):8602-8608.
[DOI] -
106. Sun J, Ahn H, Kang S, Ko SB, Song D, Um HA, et al. Exceptionally stable blue phosphorescent organic light-emitting diodes. Nat Photonics. 2022;16:212-218.
[DOI] -
107. Kim E, Park J, Jun M, Shin H, Baek J, Kim T, et al. Highly efficient and stable deep-blue organic light-emitting diode using phosphor-sensitized thermally activated delayed fluorescence. Sci Adv. 2022;8(41):eabq1641.
[DOI] -
108. Nguyen TB, Nakanotani H, Hatakeyama T, Adachi C. The role of reverse intersystem crossing using a TADF-type acceptor molecule on the device stability of exciplex-based organic light-emitting diodes. Adv Mater. 2020;32(9):1906614.
[DOI] -
109. Liu D, Yang GX, Chen Z, Xie W, Li D, Li W, et al. Highly horizontal oriented tricomponent exciplex host with multiple reverse intersystem crossing channels for high-performance narrowband electroluminescence and eye-protection white organic light-emitting diodes. Adv Mater. 2024;36(33):2403584.
[DOI] -
110. Song W, Lee JY, Cho YJ, Yu H, Aziz H, Lee KM. Electroplex as a new concept of universal host for improved efficiency and lifetime in red, yellow, green, and blue phosphorescent organic light-emitting diodes. Adv Sci. 2018;5(2):1700608.
[DOI] -
111. Jung M, Lee KH, Lee JY, Kim TY. A bipolar host based high triplet energy electroplex for an over 10 000 h lifetime in pure blue phosphorescent organic light-emitting diodes. Mater Horiz. 2020;7(2):559-565.
[DOI] -
112. Yun JH, Kim JM, Chung WJ, Lim J, Lee JY, Lee Y, et al. A novel electroplex host with dual triplet exciton up-converting channels suppressing triplet exciton induced degradation mechanisms in blue organic light-emitting diodes. J Mater Chem C. 2021;9(42):15242-15250.
[DOI] -
113. Woo JY, Park MH, Jeong SH, Kim YH, Kim BJ, Lee TW, et al. Advances in solution-processed OLEDs and their prospects for use in displays. Adv Mater. 2023;35(43):2207454.
[DOI] -
114. Kreiza G, Berenis D, Banevicius D, Jursenas S, Javorskis T, Orentas E, et al. High efficiency and extremely low roll-off solution- and vacuum-processed OLEDs based on isophthalonitrile blue TADF emitter. Chem Eng J. 2021;412:128574.
[DOI] -
115. Zhu Y, Zeng S, Gong W, Chen X, Xiao C, Ma H, et al. Molecular design of blue thermally activated delayed fluorescent emitters for high efficiency solution processable OLED via an intramolecular locking strategy. Chem Eng J. 2022;450(4):138459.
[DOI] -
116. Wada Y, Kubo S, Kaji H. Adamantyl substitution strategy for realizing solution-processable thermally stable deep-blue thermally activated delayed fluorescence materials. Adv Mater. 2018;30(8):1705641.
[DOI] -
117. Kumaresan R, Park HY, Maheshwaran A, Park H, Do Y, Song M, et al. High performance solution-processed deep-blue phosphorescence organic light-emitting diodes with EQE over 24% by employing new carbenic Ir(III) complexes. Adv Opt Mater. 2022;10(2):2101686.
[DOI] -
118. Chen L, Chang Y, Shu H, Li Q, Shi S, Wang S, et al. Achieving efficient solution-processed blue narrowband emitting OLEDs with small efficiency roll-off by using a bulky TADF sensitizer with high reverse intersystem crossing rate. Adv Opt Mater. 2023;11(2):2201898.
[DOI] -
119. Zhang K, Wang X, Chang Y, Wu Y, Wang S, Wang L. Carbazole-Decorated organoboron emitters with low-lying HOMO levels for solution-processed narrowband blue hyperfluorescence OLED devices. Angew Chem Int Ed. 2023;62(47):e202313084.
[DOI] -
120. Xie FM, An ZD, Xie M, Li YQ, Zhang GH, Zou SJ, et al. tert-Butyl substituted hetero-donor TADF compounds for efficient solution-processed non-doped blue OLEDs. J Mater Chem C. 2020;8(17):5769-5776.
[DOI] -
121. Chang YF, Yu CH, Yang SC, Hong IH, Jiang SC, Meng HF, et al. Great improvement of operation-lifetime for all-solution OLEDs with mixed hosts by blade coating. Org Electron. 2017;42:75-86.
[DOI] -
122. He Y, Qiao Z, Cai X, Li M, Li W, Xie W, Qiu W, et al. Pyridine-Based bipolar hosts for solution-processed bluish-green thermally activated delayed fluorescence devices: A subtle regulation of chemical stability and carrier transportation. ACS Appl Mater Interfaces. 2020;12(44):49905-49914.
[DOI] -
123. Liu D, He Y, Qiu W, Peng X, Li M, Li D, et al. Management of host-guest triplet exciton distribution for stable, high-efficiency, low roll-off solution-processed blue organic light-emitting diodes by employing triplet-energy-mediated hosts. Adv Funct Mater. 2023;33(32):2301327.
[DOI] -
124. Zhang X, Yan H, Zhang X, Meng H. Thermally cross-linkable hole-transport materials enable solution-processed blue OLED with LT95 over 150 h. Sci China Mater. 2024;67:2767-2777.
[DOI] -
125. Li H, Yan H, Zhang X, Shi K, Kuang C, Zheng X, et al. Highly efficient and stable solution-processed deep-blue OLEDs with LT95 over 50 h at 1000 nit. Chem Eng J. 2024;486:150142.
[DOI] -
126. Samaeifar F, Aziz H. Role of guest materials in the lower stability of solution-coated versus vacuum-deposited phosphorescent OLEDs. ACS Appl Mater Interfaces. 2022;14(6):8199-8208.
[DOI] -
127. Pu YJ, Chiba T, Ideta K, Takahashi S, Aizawa N, Hikichi T, et al. Fabrication of organic light-emitting devices comprising stacked light-emitting units by solution-based processes. Adv Mater. 2014;27(8):1327-1332.
[DOI] -
128. Niu YH, Liu MS, Ka JW, Bardeker J, Zin MT, Schofield R, et al. Crosslinkable hole-transport layer on conducting polymer for high-efficiency white polymer light-emitting diodes. Adv Mater. 2007;19(2):300-304.
[DOI] -
129. Noda H, Nakanotani H, Adachi C. Excited state engineering for efficient reverse intersystem crossing. Sci Adv. 2018;4(6):eaao6910.
[DOI] -
130. Chan CY, Tanaka M, Nakanotani H, Adachi C. Efficient and stable sky-blue delayed fluorescence organic light-emitting diodes with CIEy below 0.4. Nat Commun. 2018;9:5036.
[DOI] -
131. Cui LS, Gillett AJ, Zhang SF, Ye H, Liu Y, Chen XK, et al. Fast spin-flip enables efficient and stable organic electroluminescence from charge-transfer states. Nat Photonics. 2020;14:636-642.
[DOI]
Copyright
© The Author(s) 2025. This is an Open Access article licensed under a Creative Commons Attribution 4.0 International License (https://creativecommons.org/licenses/by/4.0/), which permits unrestricted use, sharing, adaptation, distribution and reproduction in any medium or format, for any purpose, even commercially, as long as you give appropriate credit to the original author(s) and the source, provide a link to the Creative Commons license, and indicate if changes were made.
Publisher's Note
Share And Cite