Abstract
The development of high-performance narrowband red organic light-emitting diodes (OLEDs) has garnered significant attention, offering both exciting opportunities and formidable challenges. In this study, we report the synthesis of a novel red thermally activated delayed fluorescence (TADF) molecule, 10,10',10''-([1,2,4]triazolo[1,5-a][1,3,5]triazine-2,5,7-triyltris(benzene-4,1-diyl))tris(10H-phenoxazine) (TPXZ-TAZTRZ), which integrates a highly electron-deficient triazolotriazine unit as the acceptor and three strongly electron-donating phenoxazine (PXZ) moieties as donors. TPXZ-TAZTRZ exhibits a small singlet-triplet energy gap, enabling a rapid reverse intersystem crossing rate. Additionally, it shows a broad emission spectrum peaking at 634 nm, spanning the yellow-to-red region. These features render TPXZ-TAZTRZ as an ideal TADF sensitizer for narrowband red fluorescent OLEDs. Accordingly, TPXZ-TAZTRZ was employed to sensitize the conventional fluorescent emitter DBP. The resulting TADF-sensitized fluorescence OLEDs (TSF-OLEDs) demonstrated efficient energy transfer from the TADF sensitizer to the emitter, effectively addressing the limitations previous encountered with TADF systems. The devices achieved high-performance pure red emission, with Commission International de l'Éclairage (CIE) coordinates of [0.67, 0.33], an emission peak at 612 nm, a narrow full width at half maximum (FWHM) of 27 nm, and a maximum external quantum efficiency of 16.2%.
Keywords
1. Introduction
Organic light-emitting diodes (OLEDs) are extensively utilized in applications such as flat panel displays and lighting due to their broad color spectrum, low energy consumption, and high flexibility. Achieving narrowband emission in OLEDs is essential for enhancing the accuracy and saturation of color performance and improving overall device display quality[1-3]. This challenge has prompted widespread research into various types of narrowband OLED devices, which incorporate traditional fluorescence emitters, phosphorescence emitters, and thermally activated delayed fluorescence (TADF) emitters. Among these, multiple resonance (MR) TADF materials[4-7] have shown promising results, especially in achieving high-performance blue[8-17] and green[18-27] narrowband emissions. However, compared to their blue and green OLEDs, high-performance narrowband red or pure red OLEDs remain few[28-34]. Overall, due to the constraints imposed by the bandgap law, red OLED typically require a narrower energy gap. Therefore, this requires the larger π-conjugation and stronger intramolecular charge transfer properties of red OLED, which inevitably gives rise to a large structural relaxation in the excited state (S1), along with substantial vibronic couplings between the S1 and ground (S0) states. As a result, the emission spectrum tends to broaden during electroluminescence, making it difficult to realize narrowband red emissions. Moreover, the development of red MR-TADF materials is further hampered by factors such as complex molecular architectures, lengthy and challenging synthesis routes, and generally low yields[35,36].
Traditional fluorescence materials[37,38] remain widely used in OLED applications due to their straightforward synthesis and structural diversity. For example, dibenzo{[f,f']-4,4',7,7'-tetraphenyl}diindeno[1,2,3-cd:1',2',3'-lm]perylene (DBP)[39,40] (Figure 1a), a widely adopted red dye, is favored in OLEDs for its narrowband emission and high photoluminescence quantum yield (ΦPL). However, conventional fluorescence emitters are inherently limited by a maximum internal quantum efficiency of 25%, as the remaining 75% of triplet excitons undergo non-radiative decay. To overcome these limitations, TADF-sensitized fluorescence (TSF)[41,42], also referred to as hyperfluorescence, has emerged as an effective strategy to boost OLED performance. By employing TADF materials as sensitizers to harvest triplet excitons, TSF-OLED devices exhibit improved performance[43-46]. Nevertheless, several challenges remain for DBP-based TSF-OLEDs, including low external quantum efficiency (EQE), pronounced efficiency roll-off, and inadequate color purity[40,41,47-51]. Therefore, the development of novel and highly efficient TADF sensitizers is crucial for advancing the performance of DBP-based TSF-OLED devices.
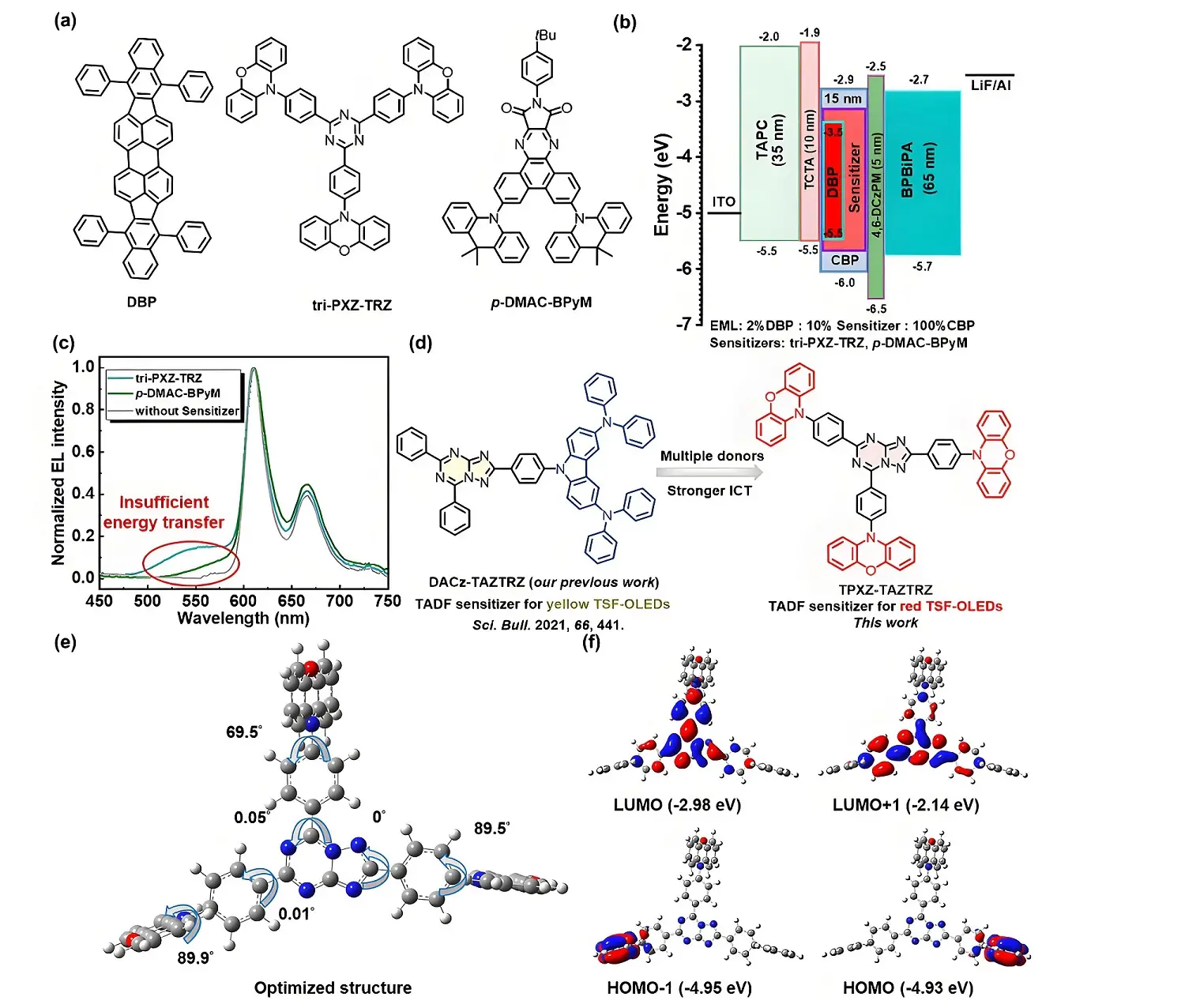
Figure 1. (a) Molecular structures of materials used in the emitting layer; (b) device structure with energy level alignment; (c) EL spectra measured at 1,000 cd m-2; (d) molecular design concept; (e) optimized structures and (f) frontier molecular orbital distributions of TPXZ-TAZTRZ. EL: electroluminescence; TPXZ-TAZTRZ: 10,10',10''-([1,2,4]triazolo[1,5-a][1,3,5]triazine-2,5,7-triyltris(benzene-4,1-diyl))tris(10H-phenoxazine); DBP: dibenzo{[f,f']-4,4',7,7'-tetraphenyl}diindeno[1,2,3-cd:1',2',3'-lm]perylene; EML: emitting layer; CBP: 4,4'-bis(9-carbazolyl)-1,1'-biphenyl; LUMO: lowest unoccupied molecular orbital; HOMO: highest occupied molecular orbital; ITO: indium tin oxide; TAPC: 1,1-bis(di-4-tolylaminophenyl)cyclohexane; TCTA: 4,4',4''-tris(carbazol-9-yl)-triphenylamine; ICT: intramolecular charge transfer.
Our initial experiments involved the fabrication of TSF-OLEDs using DBP and a previously reported tri-PXZ-TRZ sensitizer[52,53] (Figure 1a,b). The optimized device architecture consisted of the following layered structure: indium-tin-oxide (ITO)/1,1-bis((di-4-tolylamino)phenyl)-cyclohexane (TAPC) (35 nm)/4,4',4''-tris(carbazol-9-yl)-triphenylamine (TCTA) (10 nm)/emitting layer (EML) (15 nm)/4,6-bis(3-(9H-carbazol-9-yl)phenyl)pyrimidine (4,6-DCzPPM) (5 nm)/2-phenyl-1-(4-(10-(4-(2-phenyl-3a,7a-dihydro-1H-benzo[d]imidazol-1-yl)phenyl)anthracen-9-yl)phenyl)-1H-benzo[d]imidazole (BPBiPA) (65 nm)/LiF/Al. The EML comprised 2 wt% DBP, 10 wt% tri-PXZ-TRZ, 100 wt% 4,4'-bis(9-carbazolyl)-1,1'-biphenyl (CBP). The molecular structure of each functional material are illustrated in Figure S1. Although the electroluminescence (EL) spectra exhibited narrowband emission features, distinct emission peaks originating from the sensitizer were observed (Figure 1c), indicating incomplete energy transfer during the sensitization process. This shortcoming degraded the color purity of the OLEDs, as reflected by the Commission International de l'Éclairage (CIE) coordinates of [0.56, 0.39]. To address this issue, we subsequently employed an orange TADF molecule previously developed by our group as a new sensitizer[54,55]. While this adjustment improved color purity, as evidenced by a shift in the CIE coordinates to [0.63, 0.35], it still exhibited suboptimal energy transfer efficiency.
Building on these findings, we designed a highly efficient TADF sensitizer based on a triazolotriazine (TAZTRZ) acceptor, designated as TPXZ-TAZTRZ (Figure 1d). Compared to conventional triazine-based systems, triazolotriazine units feature higher electron deficiency and a flatter geometric structure, facilitating enhanced up-conversion rates and improved energy transfer. This improvements help suppresses residual sensitizer emission and enhance color purity. Additionally, the introduction of multiple donors strengthens the intermolecular charge-transfer characters, giving rise to a red TADF molecule and further enabling the red TSF-OLEDs with enhanced color purity.
2. Materials and Methods
2.1 Synthesis
Commercially available chemical reagents were used received without any further purification, unless otherwise specified. Solvents were dried and purified using an Innovative Technology PS-MD-5 solvent purification system. All reactions were conducted under a nitrogen atmosphere employing standard Schlenk techniques.
2.2 Measurements
High-resolution mass spectra were acquired using a Shimadzu LCMS-IT-TOF equipped with electrospray ionization. Nuclear magnetic resonance (NMR) spectra were recorded on an Agilent 400-MR DD2 spectrometer. Chemical shifts for the 1H NMR (400 MHz) were referenced to CDCl3 (δ = 7.26 ppm), and those for 13C NMR (100 MHz) were referenced to CDCl3 (δ = 77.16 ppm). Fluorescence spectra and photoluminescence quantum yields were measured using a Horiba Jobin Yvon-Edison Fluoromax-3 fluorescence spectrometer equipped with a calibrated integrating sphere system. Phosphorescence spectra were obtained using both a HITACHI F-7100 fluorescence spectrophotometer and the Fluoromax-3. Transient photoluminescence (PL) decay curves were recorded using a Horiba FluoroHub single-photon counting controller in combination with a Horiba TBX picosecond photon detector. Cyclic voltammetry measurements were carried out using an LK2005A electrochemical 0.1 M tetrabutylammonium hexafluorophosphate (Bu4NPF6) in CH2Cl2 as the electrolyte. A ferrocene/ferrocenium (Fc/Fc+) redox couple was used as the internalreference. A three-electrode configuration was employed, consisting of a silver/silver chloride (Ag/Ag+) reference electrode, a platinum wire, a counter electrode, and a glassy carbon working electrode. Thermogravimetric analysis (TGA) was performed on a DTG-60(H) instrument under a nitrogen atmosphere at a heating rate of 10 ºC min-1.
2.3 OLED fabrication and characterization
ITO-coated glass substrates with a sheet resistance of 15 Ω/sq were used as the anode. The ITO substrates were cleaned using an alkaline detergent, followed by sequential treatments including boiling in deionized water, ultrasonication in deionized water, oven drying, and a 10-minute oxygen plasma treatment to enhance the surface work function. Organic layers were deposited at a rate of 0.1 nm s-1 in a high-vacuum chamber maintained below 2.0 × 10-4 Pa within an inert gas glovebox. Doped and co-doped layers were formed via co-evaporating of the dopant and host materials from separate sources, with doping concentrations by adjusting the respective evaporation rates. Current density-voltage-luminance (J-V-L) characteristics were measured using a KEYSIGHT B1500A semiconductor parameter analyzer. Luminance and electroluminescence spectra were recorded using a DLM-100Z photometer and an OPT2000 spectrophotometer.
3. Results and Discussion
3.1 Theoretical calculations
Density functional theory (DFT) and time-dependent DFT calculations were first performed to investigate the ground- and excited-state structures of the TPXZ-TAZTRZ molecule. The optimized geometry (Figure 1e) reveals that the benzene bridge and the TAZTRZ unit lie nearly in the same plane with small dihedral angles, likely due to intermolecular hydrogen bonding interactions. Additionally, the dihedral angles between the phenoxazine (PXZ) donors and theiradjacent benzene bridges are 69.5°, 89.5°, and 89.9°, placing the donor and acceptor moieties in a nearly orthogonal configuration. The frontier molecular orbitals (FMOs) were further analyzed, as illustrated in Figure 1f. The highest occupied molecular orbitals (HOMO) and the next lowest (HOMO-1) are primarily located on the two separate PXZ donors with close energy levels of -4.93 eV and -4.95 eV respectively. In contrast, the lowest unoccupied molecular orbitals (LUMO) and the LUMO+1 are mainly distributed over the TAZTRZ acceptor and extend partially onto the π-bridge. Furthermore, the overlap integral of the FMOs was calculated to be 0.03877, indicating a relatively a relatively small singlet-triplet energy gap (∆EST). The spatial separation between the HOMO and LUMO, together with the small FMO overlap, is favorable for achieving a low ∆EST and promoting an efficient up-conversion process.
3.2 Synthesis
The synthesis route for TPXZ-TAZTRZ is outlined in Figure 2. The process began with a nucleophilic substitution reaction between commercially available cyanuric chloride (1) and (4-bromophenyl) magnesium iodide Grignard reagent (2), yielding intermediate 3. A second nucleophilic substitution with hydrazine monohydrate afforded compound 4. Compound 4 then underwent dehydration followed by condensation with para -bromobenzaldehyde to give compound 5. Intramolecular oxidative cyclization was subsequently performed to generate compound 6. A Dimroth rearrangement was employed to convert compound 6 into compound 7. Finally, Buchwald-Hartwig amination with multiple PXZ donor units afforded the target molecule, TPXZ-TAZTRZ. More information about the synthesis route is available in Figure S2,S3,S4,S5,S6,S7.
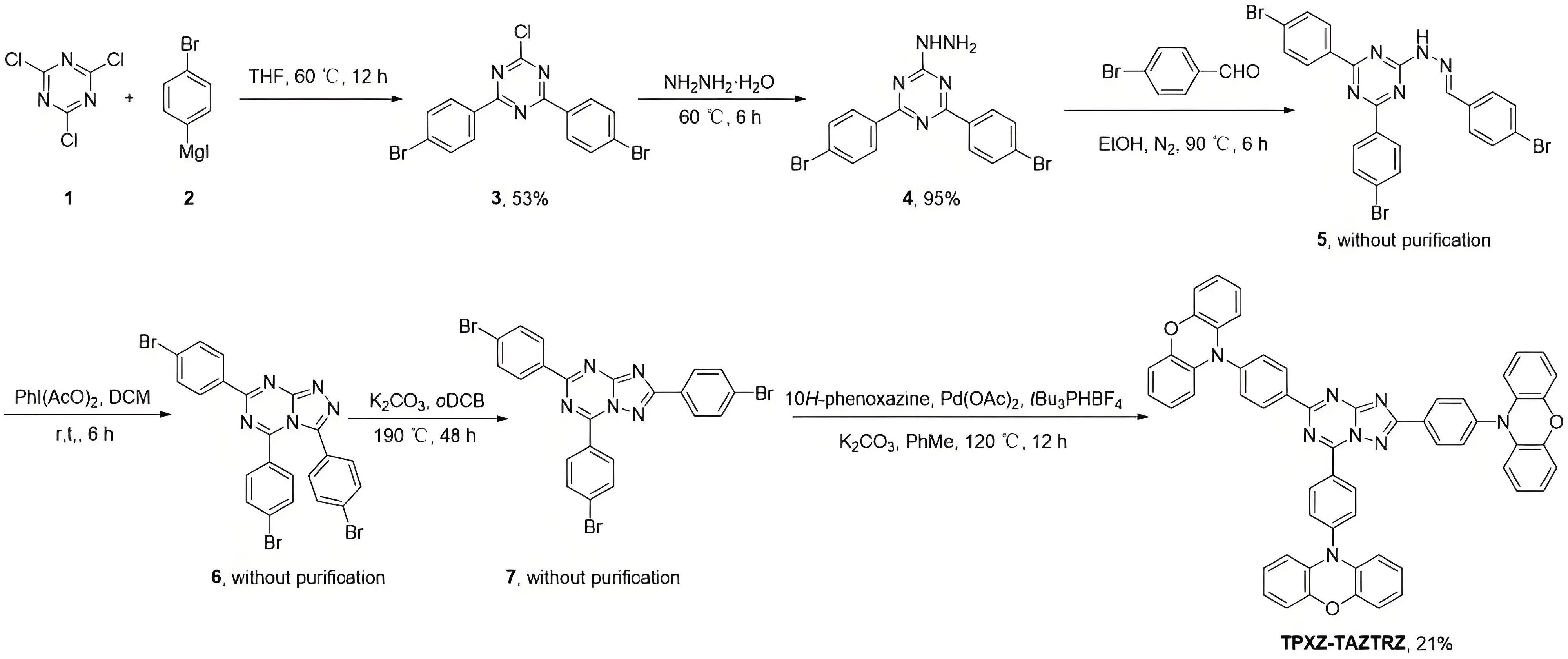
Figure 2. Synthetic route to TPXZ-TAZTRZ. TPXZ-TAZTRZ: 10,10',10''-([1,2,4]triazolo[1,5-a][1,3,5]triazine-2,5,7-triyltris(benzene-4,1-diyl))tris(10H-phenoxazine).
3.3 Photophysical properties
The PL characteristics of TPXZ-TAZTRZ are show in Figure 3a and summarized in Table 1. TPXZ-TAZTRZ exhibits a sharp and intense absorption peaks at 326 nm in the ultraviolet region, attributed to an intramolecular localized excited transition. A broad and weaker absorption band centered at 458 nm indicates the presence of an intramolecular charge transfer (CT) transition. The room-temperature fluorescence spectrum of TPXZ-TAZTRZ in toluene displays a single broad emission peak at 634 nm, further confirming its predominantly CT emission character. Notably, the phosphorescence spectrum at 77 K shows a blue shift compared to the room-temperature fluorescence spectrum, likely due to geometric differences between the singlet and triplet excited states, which influence the energy levels under different thermal conditions. To explore this effect, we also recorded the low-temperature fluorescence spectrum of TPXZ-TAZTRZ in toluene at 77 K (Figure S8). Both the fluorescence and phosphorescence spectra at low temperature revealed an extremely small singlet–triplet energy gap (∆EST). Moreover, the low-temperature fluorescence spectrum exhibited a pronounced blue shift relative to its room-temperature counterpart, possibly resulting from suppressed vibration at 77 K, in which leads to a small Stokes shift and blue shift emission. Thesinglet and triplet energy levels, estimated from the onset wavelengths of the fluorescence and phosphorescence spectra, were 2.53 eV and 2.51 eV, respectively, yielding a very small energy gap (∆EST) of 0.02 eV. Such small measured values also indicate the rationality of theoretical analysis. This minimal ∆EST facilitates efficient reverse intersystem crossing processes (RISC), supported by computational findings (Figure 1f). As shown in Figure 3b, TPXZ-TAZTRZ exhibits pronounced TADF behavior in a CBP host matrix, with a prompt fluorescence lifetime (τp) of 24.1 ns and a delayed fluorescence lifetime (τd) of 1.2 μs. In addition, TPXZ-TAZTRZ achieves a high ΦPL of 68%. Accordingly, the radiative transition rate (kR) and RISC rate (kRISC) for TPXZ-TAZTRZ are calculated to be 1.9 × 107 s-1 and 7.6 × 105 s-1, respectively, as detailed in Table 1.
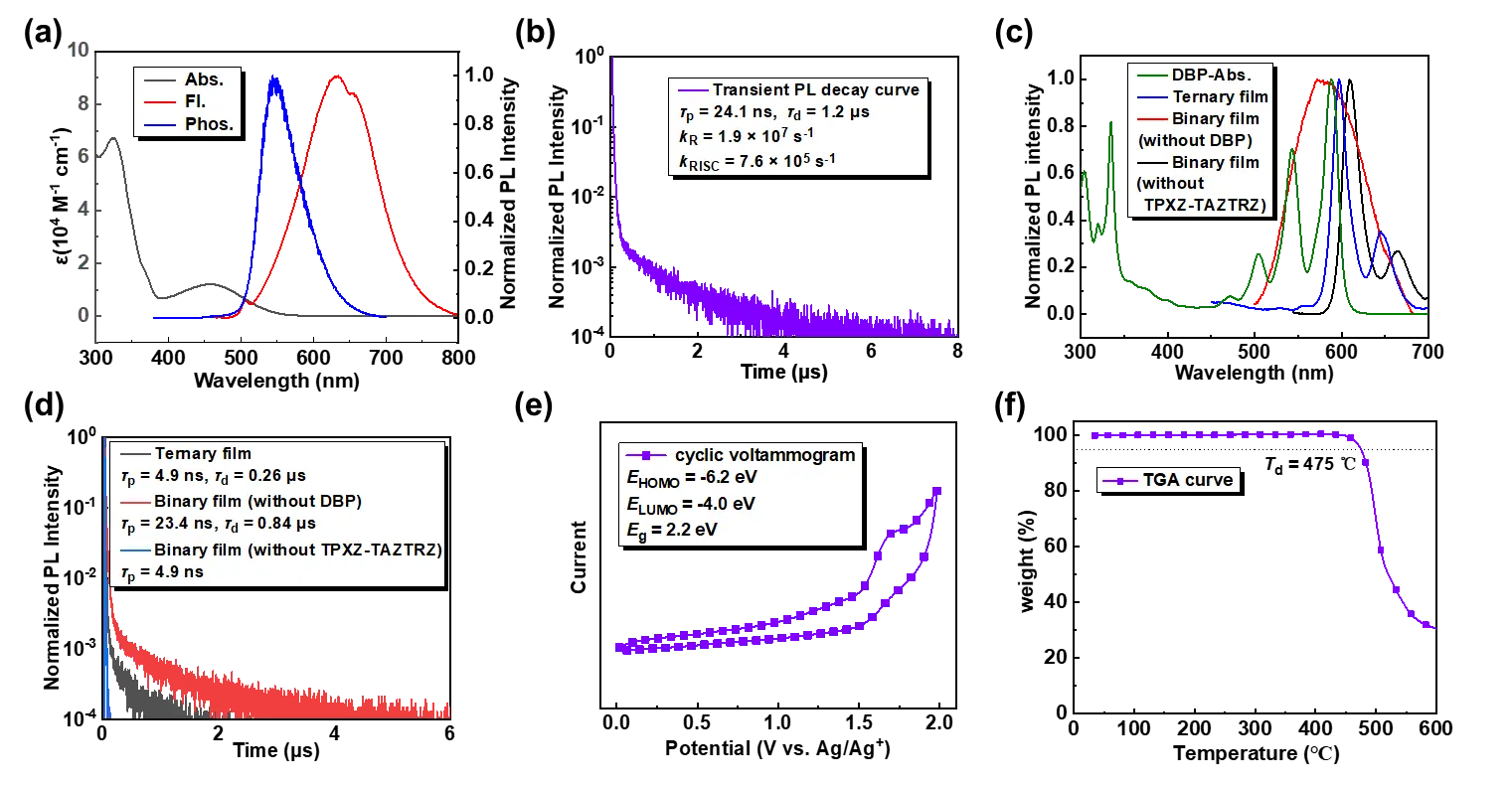
Figure 3. (a) Absorption spectra, normalized fluorescence spectra at room temperature, and phosphorescence spectra at 77K of TPXZ-TAZTRZ in toluene solution at 1.0 × 10-5 mol L-1; (b) transient PL decay curve of TPXZ-TAZTRZ in 5 wt% film in CBP; (c) normalized absorption spectrum of DBP and normalized fluorescence spectrum of TPXZ-TAZTRZ; (d) transient PL decay curves of ternary and binary films; (e) cyclic voltammogram of TPXZ-TAZTRZ in dry CH2Cl2 solution at 1.0 × 10-3 mol L-1; (f) thermogravimetric analysis curve of TPXZ-TAZTRZ. Abs.: absorption spectra; Fl.: fluorescence spectra; Phos.: phosphorescence spectra; PL: photoluminescence; TPXZ-TAZTRZ: 10,10',10''-([1,2,4]triazolo[1,5-a][1,3,5]triazine-2,5,7-triyltris(benzene-4,1-diyl))tris(10H-phenoxazine); CBP: 4,4'-bis(9-carbazolyl)-1,1'-biphenyl; DBP: dibenzo{[f,f']-4,4',7,7'-tetraphenyl}diindeno[1,2,3-cd:1',2',3'-lm]perylene; LUMO: lowest unoccupied molecular orbital; HOMO: highest occupied molecular orbital; TGA: thermogravimetric analysis.
Compound | Td1 [ºC] | HOMO2 [eV] | LUMO3 [eV] | λabs [nm] | λFL [nm] | ES1 [eV] | ET1 [eV] |
TPXZ-TAZTRZ | 475 | -6.2 | -4.0 | 326, 458 | 634 | 2.53 | 2.51 |
Compound | ΔEST [eV] | ΦPL4 [%] | τp4 [ns] | τd4 [μs] | C25 [%] | kR6 [107 s-1] | kRISC7 [105 s-1] |
TPXZ-TAZTRZ | 0.02 | 68 | 24.1 | 1.2 | 33.2 | 1.9 | 7.6 |
1Deposition temperature with 5% weight loss calculated from thermogravimetric analysis curve (Figure 2f); 2calculated from cyclic voltammogram curve (Figure 2e). EHOMO = -4.8 - (EOX - EFe,1/2) (eV); 3calculated from the HOMO energy level and the energy gap from the absorption spectrum. ELUMO = Eg + EHOMO (eV); 4measured in CBP host at 5 wt%; 5proportion of delayed component; 6kR = C1ΦPL/τp; 7kRISC = C2/[C1τd(1-ΦPLC1)]; TPXZ-TAZTRZ: 10,10',10''-([1,2,4]triazolo[1,5-a][1,3,5]triazine-2,5,7-triyltris(benzene-4,1-diyl))tris(10H-phenoxazine); LUMO: lowest unoccupied molecular orbital; HOMO: highest occupied molecular orbital.
Subsequently, we evaluated the potential of TPXZ-TAZTRZ as a sensitizer for DBP. As shown in Figure 3c, the absorption spectra of DBP exhibits significant spectral overlap with the PL spectrum of TPXZ-TAZTRZ-based binary film (10 wt% TPXZ-TAZTRZ in CBP, excitation wavelength: 380 nm). This spectral overlap indicates that TPXZ-TAZTRZ is a suitable sensitizer for DBP, which is crucial for efficient Förster energy transfer (FET) during the sensitization process. Furthermore, the ternary film (excitation wavelength: 440 nm), containing 2% DBP and 10% TPXZ-TAZTRZ in a CBP matrix, for showcased narrowband red emission predominantly from DBP, with an emission peak at 597 nm and full width at half maximum (FWHM) values of 20 nm, demonstrating the effective FET process between TPXZ-TAZTRZ sensitizer and DBP emitter. According to the transient PL decay curve (Figure 3d), the rates of FET (kFET) and dexter energy transfer (DET) rates (kDET) for the ternary film were calculated and are summarized in Table S1. The fast kFET (~ 108 s-1) and relatively slow kDET (~ 106 s-1) promote the TSF process, contributing to the excellent color purity of the high-performance TSF-OLEDs. Additionally, the introduction of DBP reduces the exciton lifetime of the sensitizer due to energy transfer between the sensitizer and the emitter. This creates additional exciton decay pathways, ultimately shortening the overall exciton lifetime. The Förster radium (R0) between the TPXZ-TAZTRZ sensitizer and the DBP emitter was estimated to be 34 nm, which is consistent with the characteristics of efficient Förster energy transfer, as shown in Table S2.
Prior to device fabrication, the HOMO and LUMO energy levels of TPXZ-TAZTRZ were determined from cyclic voltammetry measurements and the optical energy gap. The HOMO and LUMO levels were found to be -6.2 eV and -4.0 eV, respectively (Figure 3e, Table 1). In addition, TPXZ-TAZTRZ exhibited excellent thermal stability, as evidenced by a smooth TGA curve with a deposition temperature (corresponding to 5% weight loss) of 475 °C, as shown in Figure 3f.
3.4 EL properties
Finally, TSF-OLEDs based on DBP were fabricated using the mentioned structure (Figure 1b). In these devices, the EML comprised 2 wt% DBP, 10 wt% TPXZ-TAZTRZ, and 100 wt% CBP. The device performance is presented in Figure 4 and summarized in Table 2. As shown in the EL spectra (Figure 4a), the TSF-OLEDs exhibit no emission from the TADF sensitizer, indicating efficient energy transfer from the sensitizer to the emitter. The corresponding CIE chromaticity coordinates are [0.666, 0.332] (Figure 3b), closely matching the standard red defined by the NTSC, demonstrating the device's potential for display applications. Furthermore, the TSF-OLEDs exhibit excellent device performance, achieving a maximum EQE (EQE max) of 16.2%, which is significantly higher than the 3.0% EQEmax observed in OLEDs without the TADF sensitizer. In addition, the introduction of sensitizers in TSF-OLEDs reduces turn-on voltage (V on) from 3.7 V (without TPXZ-TAZTRZ) to 3.2 V (with TPXZ-TAZTRZ), and increases the maximum luminance (L max) from 2,426 cd/m2 to 12,276 cd/m2. However, the TSF-OLEDs exhibit severe efficiency roll-off, which may be attributed to the rigidity of the DBP emitter or an inadequate device structure. These issues may be addressed through the screening of alternative functional layer materials and the optimization of device architecture.
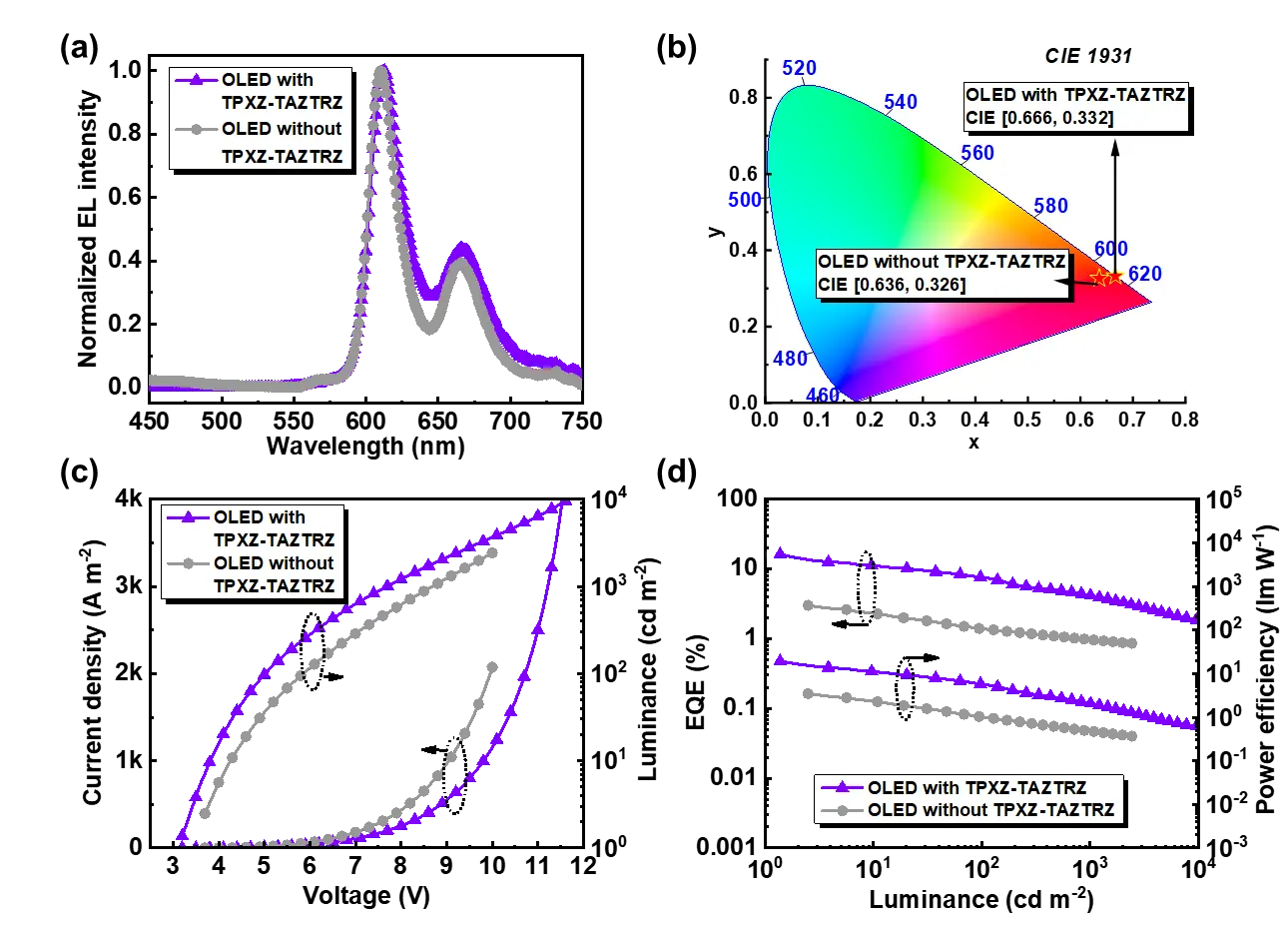
Figure 4. (a) EL spectra and (b) colour coordinates measured at 1,000 cd m-2; (c) current density-voltage and luminance-voltage characteristics; (d) EQE-luminance and power efficiency-luminance curves of OLEDs. EL: electroluminescence; OLEDs: organic light-emitting diodes; EQE: external quantum efficiency; TPXZ-TAZTRZ: 10,10',10''-([1,2,4]triazolo[1,5-a][1,3,5]triazine-2,5,7-triyltris(benzene-4,1-diyl))tris(10H-phenoxazine); CIE: Commission International de l'Éclairage.
Device | λEL [nm] | FWHM [nm] | CIE [x, y] | EQEmax [%] | EQE100 [%] | EQE1000 [%] | Von [V] | Lummax [cd m-2 ] |
TSF-OLED | 612 | 27 | [0.67, 0.33] | 16.2 | 7.6 | 4.2 | 3.2 | 12,276 |
non TSF-OLED | 610 | 25 | [0.64, 0.33] | 3.0 | 1.4 | 1.0 | 3.7 | 2,426 |
EL: electroluminescence; OLED: organic light-emitting diode; TSF-OLED: TADF-sensitized fluorescence OLED; CIE: Commission International de l'Éclairage; EQE: external quantum efficiency.
4. Conclusion
In summary, we have developed a novel red TADF molecule, TPXZ-TAZTRZ, which incorporates a highly electron-deficient TAZTRZ acceptor flanked by three strong electron-donating PXZ donors. TPXZ-TAZTRZ exhibits a fast RISC rate and significant spectral overlap with the narrowband red emitter DBP. As a result, TSF-OLEDs employing TPXZ-TAZTRZ as the sensitizer and DBP as the emitter demonstrated efficient energy transfer from the TADF sensitizer to the emitter, leading to pure red emission. The devices achieved CIE coordinates of [0.67, 0.33], an emission peak at 612 nm with a narrow FWHM of 27 nm, and a EQEmax of 16.2%. This study not only presents a promising TADF sensitizer for high-performance red TSF-OLEDs, but also highlights the critical role of a high RISC rate in facilitating efficient energy transfer from the sensitizer to the emitter.
Supplementary materials
The supplementary material for this article is available at: Supplementary materials.
Authors contribution
Tian Y, Zhang J: Performed the synthesis and characterization of the organic compounds, conducted OLEDs investigation, wrote the manuscript.
Bin Z: Supervised the project, wrote the manuscript.
Conflict of interest
The authors declare no conflict of interest.
Ethical approval and consent
Not applicable.
Availability of data and materials
The data and materials could be obtained from the corresponding author upon request.
Funding
This work was financially supported by the National NSF of China (No. 22275127).
Copyright
© The Author(s) 2025.
References
-
1. Fleetham T, Li G, Wen L, Li J. Efficient "pure" blue OLEDs employing tetradentate Pt complexes with a narrow spectral bandwidth. Adv Mater. 2014;26(41):7116-7121.
[DOI] -
2. Liu Y, Li C, Ren Z, Yan S, Bryce MR. All-organic thermally activated delayed fluorescence materials for organic light-emitting diodes. Nat Rev Mater. 2018;3(4):18020.
[DOI] -
3. Hong G, Gan X, Leonhardt C, Zhang Z, Seibert J, Busch JM, et al. A brief history of OLEDs—emitter development and industry milestones. Adv Mater. 2021;33(9):2005630.
[DOI] -
4. Hatakeyama T, Shiren K, Nakajima K, Nomura S, Nakatsuka S, Kinoshita K, et al. Ultrapure blue thermally activated delayed fluorescence molecules: efficient HOMO-LUMO separation by the multiple resonance effect. Adv Mater. 2016;28(14):2777-2781.
[DOI] -
5. Matsui K, Oda S, Yoshiura K, Nakajima K, Yasuda N, Hatakeyama T. One-shot multiple borylation toward BN-doped nanographenes. J Am Chem Soc. 2017;140(4):1195-1198.
[DOI] -
6. Kondo Y, Yoshiura K, Kitera S, Nishi H, Oda S, Gotoh H, et al. Narrowband deep-blue organic light-emitting diode featuring an organoboron-based emitter. Nat Photonics. 2019;13(10):678-682.
[DOI] -
7. Ikeda N, Oda S, Matsumoto R, Yoshioka M, Fukushima D, Yoshiura K, et al. Solution-processable pure green thermally activated delayed fluorescence emitter based on the multiple resonance effect. Adv Mater. 2020;32(40):2004072.
[DOI] -
8. Suresh SM, Duda E, Hall D, Yao Z, Bagnich S, Slawin AMZ, et al. A deep blue B,N-doped heptacene emitter that shows both thermally activated delayed fluorescence and delayed fluorescence by triplet-triplet annihilation. J Am Chem Soc. 2020;142(14):6588-6599.
[DOI] -
9. Oda S, Kawakami B, Yamasaki Y, Matsumoto R, Yoshioka M, Fukushima D, et al. One-shot synthesis of expanded heterohelicene exhibiting narrowband thermally activated delayed fluorescence. J Am Chem Soc. 2021;144(1):106-112.
[DOI] -
10. Bian J, Chen S, Qiu L, Tian R, Man Y, Wang Y, et al. Ambipolar self-host functionalization accelerates blue multi-resonance thermally activated delayed fluorescence with internal quantum efficiency of 100%. Adv Mater. 2022;34(17):2110547.
[DOI] -
11. Fan T, Zhang Y, Wang L, Wang Q, Yin C, Du M, et al. One-shot synthesis of B/N-doped calix[4].arene exhibiting narrowband multiple resonance fluorescence. Angew Chem Int Ed. 2022;61(52):e202213585.
[DOI] -
12. Lv X, Miao J, Liu M, Peng Q, Zhong C, Hu Y, et al. Extending the π-skeleton of multi-resonance TADF materials towards high-efficiency narrowband deep-blue emission. Angew Chem Int Ed. 2022;61(29):e202201588.
[DOI] -
13. Park IS, Yang M, Shibata H, Amanokura N, Yasuda T. Achieving ultimate narrowband and ultrapure blue organic light-emitting diodes based on polycyclo-heteraborin multi-resonance delayed-fluorescence emitters. Adv Mater. 2022;34(9):2107951.
[DOI] -
14. Huang F, Fan XC, Cheng YC, Wu H, Xiong X, Yu J, et al. Combining carbazole building blocks and ν-DABNA heteroatom alignment for a double boron-embedded MR-TADF emitter with improved performance. Angew Chem Int Ed. 2023;62(32):e202306413.
[DOI] -
15. Lei B, Huang Z, Li S, Liu J, Bin Z, You J. Medium-ring strategy enables multiple resonance emitters with twisted geometry and fast spin-flip to suppress efficiency roll-off. Angew Chem Int Ed. 2023;62(12):e202218405.
[DOI] -
16. Meng G, Zhou J, Huang T, Dai H, Li X, Jia X, et al. B-N/B-O contained heterocycles as fusion locker in multi-resonance frameworks towards highly-efficient and stable ultra-narrowband emission. Angew Chem Int Ed. 2023;62(45):e202309923.
[DOI] -
17. Tian Y, Su R, Liu J, Lei B, Liu M, Bin Z. Blue multiple resonance emitters exhibiting fast spin flip. Sci China Mater. 2024;67(7):2311-2318.
[DOI] -
18. Xu Y, Li C, Li Z, Wang Q, Cai X, Wei J, et al. Constructing charge-transfer excited states based on frontier molecular orbital engineering: narrowband green electroluminescence with high color purity and efficiency. Angew Chem Int Ed. 2020;59(40):17442-17446.
[DOI] -
19. Xu Y, Wang Q, Cai X, Li C, Wang Y. Highly efficient electroluminescence from narrowband green circularly polarized multiple resonance thermally activated delayed fluorescence enantiomers. Adv Mater. 2021;33(21):2100652.
[DOI] -
20. Zhang Y, Wei J, Zhang D, Yin C, Li G, Liu Z, et al. Sterically wrapped multiple resonance fluorophors for suppression of concentration quenching and spectrum broadening. Angew Chem Int Ed. 2021;61(2):e202113206.
[DOI] -
21. Hu YX, Miao J, Hua T, Huang Z, Qi Y, Zou Y, et al. Efficient selenium-integrated TADF OLEDs with reduced roll-off. Nat Photonics. 2022;16(11):803-810.
[DOI] -
22. Liu Y, Xiao X, Huang Z, Yang DZ, Ma D, Liu J, et al. Space-confined donor-acceptor strategy enables fast spin-flip of multiple resonance emitters for suppressing efficiency roll-off. Angew Chem Int Ed. 2022;61(40):e202210210.
[DOI] -
23. Uemura S, Oda S, Hayakawa M, Kawasumi R, Ikeda N, Lee YT, et al. Sequential multiple borylation toward an ultrapure green thermally activated delayed fluorescence material. J Am Chem Soc. 2022;145(3):1505-1511.
[DOI] -
24. Xu Y, Wang Q, Wei J, Peng X, Xue J, Wang Z, et al. Constructing organic electroluminescent material with very high color purity and efficiency based on polycyclization of the multiple resonance parent core. Angew Chem Int Ed. 2022;61(30):e202204652.
[DOI] -
25. Cheng H, Lan J, Yang Y, Bin Z. Spirobifluorene-fused strategy enables pure-green multiple resonance emitters with low efficiency roll-off. Mater Horiz. 2024;11(19):4674-4680.
[DOI] -
26. Li Z, Li Z, Zhang S, Liu M, Gao G, You J, et al. Narrowband pure-green emitters based on naphthalene-fused meta-positioned double boron framework. Sci China Mater. 2024;67(5):1581-1587.
[DOI] -
27. Wu ZG, Xin Y, Lu C, Huang W, Xu H, Liang X, et al. Precise regulation of multiple resonance distribution regions of a B,N-embedded polycyclic aromatic hydrocarbon to customize its BT2020 green emission. Angew Chem Int Ed. 2024;63(7):e202318742.
[DOI] -
28. Yang M, Park IS, Yasuda T. Full-color, narrowband, and high-efficiency electroluminescence from boron and carbazole embedded polycyclic heteroaromatics. J Am Chem Soc. 2020;142(46):19468-19472.
[DOI] -
29. Liu Y, Xiao X, Ran Y, Bin Z, You J. Molecular design of thermally activated delayed fluorescent emitters for narrowband orange-red OLEDs boosted by a cyano-functionalization strategy. Chem Sci. 2021;12(27):9408-9412.
[DOI] -
30. Cai X, Xu Y, Pan Y, Li L, Pu Y, Zhuang X, et al. Solution-processable pure-red multiple resonance-induced thermally activated delayed fluorescence emitter for organic light-emitting diode with external quantum efficiency over 20%. J Am Chem Soc. 2023;62(7):e202216473.
[DOI] -
31. Fan XC, Huang F, Wu H, Wang H, Cheng YC, Yu J, et al. A quadruple-borylated multiple-resonance emitter with para/meta heteroatomic patterns for narrowband orange-red emission. Angew Chem Int Ed. 2023;62(35):e202305580.
[DOI] -
32. Hu Y, Huang M, Liu H, Miao J, Yang C. Narrowband fluorescent emitters based on BN-doped polycyclic aromatic hydrocarbons for efficient and stable organic light-emitting diodes. Angew Chem Int Ed. 2023;62(46):e202312666.
[DOI] -
33. Meng G, Zhou J, Han XS, Zhao W, Zhang Y, Li M, et al. B-N covalent bond embedded double hetero-[n].helicenes for pure red narrowband circularly polarized electroluminescence with high efficiency and stability. Adv Mater. 2024;36(5):2307420.
[DOI] -
34. Luo A, Bao Y, Liu J, Yang Y, Deng Y, You J, et al. Design of thermally activated delayed fluorescence materials: transition from carbonyl to amide-based acceptors. Angew Chem Int Ed. 2024;63:e202411464.
[DOI] -
35. Zhang Y, Zhang D, Huang T, Gillett AJ, Liu Y, Hu D, et al. Multi-resonance deep-red emitters with shallow potential-energy surfaces to surpass energy-gap law. Angew Chem Int Ed. 2021;60(37):20498-20503.
[DOI] -
36. Zou Y, Hu J, Yu M, Miao J, Xie Z, Qiu Y, et al. High-performance narrowband pure-red OLEDs with external quantum efficiencies up to 36.1% and ultralow efficiency roll-off. Adv Mater. 2022;34(29):2201442.
[DOI] -
37. Ha JM, Hur SH, Pathak A, Jeong JE, Woo HY. Recent advances in organic luminescent materials with narrowband emission. NPG Asia Mater. 2021;13(1):53.
[DOI] -
38. Luo A, Bao Y, Liu X, Liu J, Han W, Yang G, et al. Unlocking structurally nontraditional naphthyridine-based electron-transporting materials with C-H activation-annulation. J Am Chem Soc. 2024;146(9):6240-6251.
[DOI] -
39. Li Y, Sheriff HKM, Liu X, Wang CK, Ding K, Han H, et al. Vacuum-deposited biternary organic photovoltaics. J Am Chem Soc. 2019;141(45):18204-18210.
[DOI] -
40. Li Z, Hu X, Liu G, Tian L, Gao H, Dong X, et al. High-efficiency red-fluorescent organic light-emitting diodes with excellent color purity. J Phys Chem C. 2021;125(3):1980-1989.
[DOI] -
41. Nakanotani H, Higuchi T, Furukawa T, Masui K, Morimoto K, Numata M, et al. High-efficiency organic light-emitting diodes with fluorescent emitters. Nat Commun. 2014;5(1):4016.
[DOI] -
42. Zhang D, Duan L, Li C, Li Y, Li H, Zhang D, et al. High-efficiency fluorescent organic light-emitting devices using sensitizing hosts with a small singlet-triplet exchange energy. Adv Mater. 2014;26(29):5050-5055.
[DOI] -
43. Wang F, Zhang L, Han W, Bin Z, You J. Intramolecular C-H activation as an easy toolbox to synthesize pyridine-fused bipolar hosts for blue organic light-emitting diodes. Angew Chem Int Ed. 2022;61(32):e202205380.
[DOI] -
44. Han W, Liu J, Ran C, Huang Z, Gao G, You J, et al. Alignment of heptagonal diimide and triazine enables narrowband pure-blue organic light-emitting diodes with low efficiency roll-off. Angew Chem Int Ed. 2023;62(48):e202312297.
[DOI] -
45. Liu J, Liu J, Li H, Bin Z, You J. Boron-dipyrromethene-based fluorescent emitters enable high-performance narrowband red organic light-emitting diodes. Angew Chem Int Ed. 2023;62(31):e202306471.
[DOI] -
46. Wu Y, Liu X, Liu J, Yang G, Deng Y, Bin Z, et al. Nitrogen effects endowed by doping electron-withdrawing nitrogen atoms into polycyclic aromatic hydrocarbon fluorescence emitters. J Am Chem Soc. 2024;146(23):15977-15985.
[DOI] -
47. Ferschke T, Hofmann A, Brütting W, Pflaum J. Application of fluorescent molecules as noninvasive sensors for optoelectronic characterization on nanometer length scales. ACS Appl Electron Mater. 2019;2(1):186-194.
[DOI] -
48. Shin DJ, Kim SC, Lee JY. A reverse intersystem crossing managing assistant dopant for high external quantum efficiency red organic light-emitting diodes. J Mater Chem C. 2022;10(12):4821-4830.
[DOI] -
49. Lee J, Jo U, Lee JY. Suppression of dexter energy transfer through modulating donor segments of thermally activated delayed fluorescence assistant dopants. ACS Appl Mater Interfaces. 2023;15(17):21261-21269.
[DOI] -
50. Zheng YQ, Yu JL, Wang C, Yang F, Wei B, Zhang JH, et al. Highly efficient red fluorescent organic light-emitting diodes by sorbitol-doped PEDOT:PSS. J Phys D Appl Phys. 2018;51(22):225302.
[DOI] -
51. Chen D, Cai X, Li XL, He Z, Cai C, Chen D, et al. Efficient solution-processed red all-fluorescent organic light-emitting diodes employing thermally activated delayed fluorescence materials as assistant hosts: molecular design strategy and exciton dynamic analysis. J Mater Chem C. 2017;5(21):5223-5231.
[DOI] -
52. Tanaka H, Shizu K, Nakanotani H, Adachi C. Twisted intramolecular charge transfer state for long-wavelength thermally activated delayed fluorescence. Chem Mater. 2013;25(18):3766-3771.
[DOI] -
53. Liu J, Bin Z, Yang D, Ma D, You J. Triazolotriazine-based mixed host for pure-red phosphorescent organic light-emitting diodes exhibiting ultra-low efficiency roll-off. Chem Eng J. 2023;466:142910.
[DOI] -
54. Su R, Zhao Y, Yang F, Duan L, Lan J, Bin Z, et al. Triazolotriazine-based thermally activated delayed fluorescence materials for highly efficient fluorescent organic light-emitting diodes (TSF-OLEDs). Sci Bull. 2021;66(5):441-448.
[DOI] -
55. Hu Y, Zhang Y, Han W, Li J, Pu X, Wu D, et al. Orange-red organic light emitting diodes with high efficiency and low efficiency roll-off: boosted by a fused acceptor composed of pyrazine and maleimide. Chem Eng J. 2022;428:131186.
[DOI]
Copyright
© The Author(s) 2025. This is an Open Access article licensed under a Creative Commons Attribution 4.0 International License (https://creativecommons.org/licenses/by/4.0/), which permits unrestricted use, sharing, adaptation, distribution and reproduction in any medium or format, for any purpose, even commercially, as long as you give appropriate credit to the original author(s) and the source, provide a link to the Creative Commons license, and indicate if changes were made.
Publisher's Note
Share And Cite