Lan-Lan Lou, Institute of New Catalytic Materials Science, School of Materials Science and Engineering, National Institute of Advanced Materials, Nankai University, Tianjin 300350, China. E-mail: lllou@nankai.edu.cn
Abstract
Photocatalytic reduction of CO2 to produce valuable fuels or chemicals is a promising CO2 utilization technology, which is of great significance for carbon emission reduction. The unique features of the UiO series of metal-organic frameworks (MOFs), such as the excellent water and chemical stability, notable structural tunability, broad and adjustable light-harvesting capacity, strong electron-hole separation ability, and high porosity and specific surface area, make them a class of photocatalysts with great potential for the CO2 reduction reaction (CO2RR). Significant progress has been made in the development of efficient UiO-based photocatalysts for CO2RR. This paper provides a summary of recent research advances in UiO-MOFs for photocatalytic CO2RR. The characteristics, synthesis methods, and modifications of UiO-based materials, along with their photocatalytic performance, are described. Various modification strategies for UiO-MOFs, including band-gap engineering, defect engineering, introduction of metal species, and construction of composite materials, are summarized and discussed. The challenges facing UiO-MOFs in photocatalytic CO2RR and potential future development directions are also presented. This review is intended to provide insights into CO2 photoreduction using UiO-based materials and to encourage further research and development in this promising field.
Keywords
1. Introduction
The rapid development of industry and the extensive use of fossil fuels have led to a sharp increase in greenhouse gas concentrations since the last century. CO2, the major component of greenhouse gases, accounts for approximately 72% of total emissions[1]. China’s carbon emissions have increased significantly since 2013 and are expected to reach their peak by 2030[2]. As key technologies for CO2 emission reduction, carbon capture, utilization, and storage have attracted considerable attention in recent decades. In particular, CO2 utilization, which involves converting CO2 into high-value fuels or chemicals, has been widely studied because it contributes to achieving a sustainable carbon cycle.
The conversion or catalytic transformation of CO2 into chemicals is a promising approach to utilize excess CO2. However, due to the chemical stability of CO2, its activation requires overcoming significant kinetic inertia and a high thermodynamic energy barrier, necessitating substantial energy input to break the C=O double bond. Artificial photosynthesis, an eco-friendly technology that mimics natural photosynthesis, converts light energy into chemical energy. Photocatalytic CO2 reduction is the central process in artificial photosynthesis. Solar energy is abundant, freely available, renewable, and environmentally friendly. Consequently, there has been growing interest in photocatalytic CO2 conversion. Various photocatalysts have been extensively studied over the past few decades, including metal-organic frameworks (MOFs)[3-6], ionic liquids[7,8], covalent organic frameworks[9-11], core-shell nanomaterials[12], and inorganic semiconductors such as oxides[13-15] and sulfides[16].
MOFs, an emerging class of crystalline materials with periodic porous structures, have attracted widespread attention in the field of photocatalysis[4,17-19] due to their unique properties, including broad and tunable light-harvesting capacity, strong electron-hole separation ability, highly compatible and adjustable structures, uniformly dispersed and easily accessible catalytic active sites, as well as high porosity and specific surface area (SSA). However, most MOFs still suffer from certain limitations that hinder their practical applications, such as the structure collapse under strong acidic or alkaline conditions, or at elevated temperatures and pressures, along with poor water stability, limited conductivity, and inadequate chemical stability.
The UiO series of MOFs (represents the University of Oslo) exhibit significant advantages in photocatalysis due to their remarkable stability and cycling performance. The high bond energy of the Zr-O bond (~ 800 kJ·mol-1) endows UiO-MOFs with outstanding acid resistance, water resistance, and thermal stability (up to 500 °C), which are markedly superior to those of most other MOFs[4,20-22]. In the context of photocatalytic CO2 reduction reaction (CO2RR), the tunable energy band structures of UiOs make them a promising class of photocatalysts capable of modulating product selectivity with relative ease. Consequently, UiO-based materials have been extensively studied for photocatalytic CO2RR, and substantial progress has been made in this field.
Several reviews have examined the use of UiO-66-based materials as photocatalysts for addressing environmental issues[23] and degrading aquatic pollutants[24], as well as the applications of UiO-67 in various fields[25,26]. However, there is currently no review that specifically focuses on the advances of UiO-MOFs in photocatalytic CO2RR. Therefore, this paper provides a comprehensive overview of the synthesis and modification of UiOs materials, along with recent research progress on modified UiO and their composites as photocatalysts for CO2RR. In addition, the challenges and future prospects of UiO-based photocatalysts for CO2RR are discussed.
2. Synthesis and Modification of UiO-MOFs
2.1 Synthesis of UiO-MOFs
The UiO series of MOFs are a class of porous materials constructed from Zr6O4(OH)4 secondary building unit (SBU) and various linear dicarboxylic acid ligands, such as 1,4-benzenedicarboxylic acid (H2BDC) for UiO-66, 4,4’-biphenyl dicarboxylic acid (BPDC) for UiO-67, and 4,4’-triphenyl dicarboxylic acid (TPDC) for UiO-68. These materials feature a three-dimensional porous framework composed of an octahedral central pore cage and eight surrounding tetrahedral corner cages[20].
The main synthesis methods for UiO-MOFs include solvo-/hydrothermal, microwave-assisted, mechanochemical, and electrochemical approaches[27]. Among these, the solvo-/hydrothermal method is the most commonly used and is also considered the most effective for producing high-quality UiO-MOFs. As shown in Figure 1, typical solvo-/hydrothermal synthesis involves mixing ZrCl4 or ZrClO4 with organic linkers, specifically H2BDC, BPDC and TPDC, in dimethylformamide (DMF), followed by a solvothermal reaction at a specific temperature[20,29]. The physicochemical properties of the resulting UiO materials, including morphology, particle size, and crystallinity, are significantly influenced by the reaction temperature and the addition of modulators. Although this method can yield UiO products with excellent crystallinity and well-ordered morphology, it generally requires a long time (typically 24 to 48 hours) and the use of toxic organic solvents such as DMF, along with relatively high energy consumption.
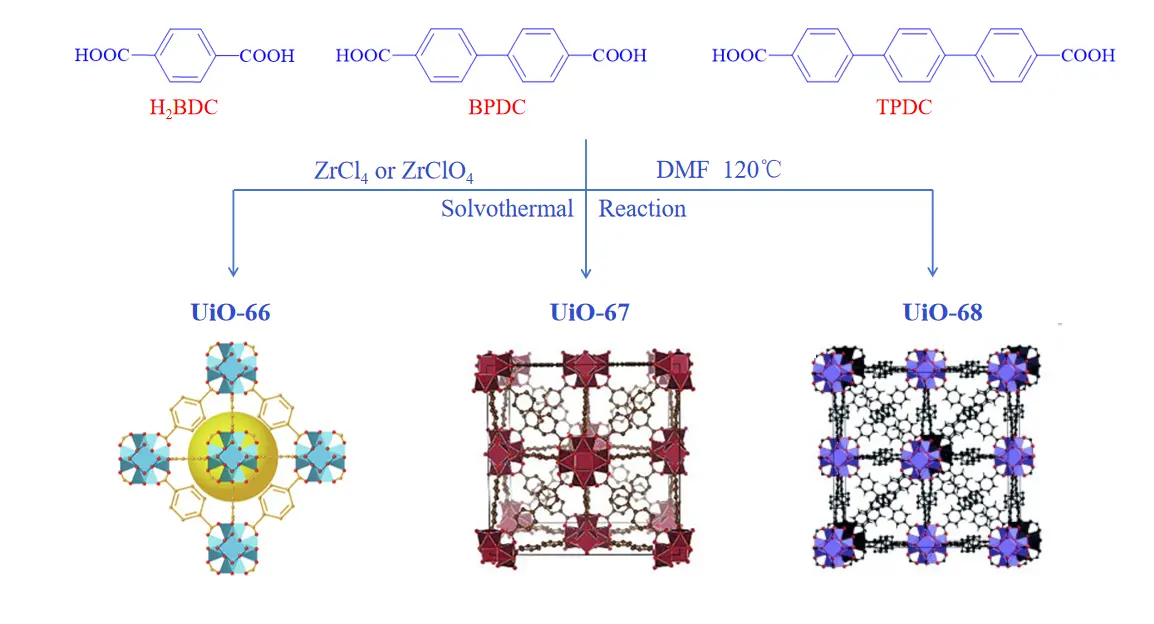
Figure 1. Synthetic routes and structure diagrams of UiO-66. Republished with permission from[23]; UiO-67. Republished with permission from[25], and UiO-68. Republished with permission from[28]. H2BDC: 1,4-benzenedicarboxylic acid; BPDC: 4,4’-biphenyl dicarboxylic acid; TPDC: 4,4’-triphenyl dicarboxylic acid.
Microwave-assisted synthesis allows precise control of reaction conditions, including temperature and pressure, promotes crystal nucleation, shortens synthesis time, and enables the production of nanoscale UiO materials[30,31]. This method is highly efficient and consumes less energy during synthesis, thereby facilitating batch production. However, it also presents challenges such as high equipment cost and limited reaction scale, which hinder its application in efficient large-scale production.
Mechanical grinding is a green synthesis method that uses little or no solvent and avoids high temperatures, strong acids, and excessive reactants, while offering high UiO yield and a short reaction time[32]. The framework is formed either through direct grinding or via spontaneous self-assembling by mixing solid reactants and exposing them to organic vapors. This method is simple, efficient, and environmentally friendly. However, the reaction rate during mechanical grinding is difficult to control, which limits the understanding of its mechanism at the molecular level. In addition, the crystallinity, porosity, and SSA of the resulting products are generally lower than those obtained by solvothermal synthesis.
The electrochemical synthesis method uses metals as the source of metal ions, thereby effectively avoiding the influence of anions (Cl-, NO3-, SO42-, etc.). Under appropriate conditions of electrolyte composition, voltage, and reaction time, UiO materials synthesized by this method exhibit notable advantages, including high purity, good porosity, and uniform morphology[33]. Additionally, it is considered one of the promising approaches for achieving continuous production of UiO-MOFs under mild reaction conditions[34]. However, this method typically results in low yields and is prone to producing by-products.
2.2 Modification of UiO-MOFs
UiO-MOFs have demonstrated considerable potential in photocatalysis. However, pristine UiO materials typically exhibit strong ultraviolet absorption but little to no visible light response, resulting in relatively poor photocatalytic activity. Through rational design and modification, key properties such as optical response range and charge separation efficiency can be significantly improved. Generally, modification strategies for UiO-MOFs fall into two main categories: de novo synthesis and post-synthetic modification (PSM).
De novo synthesis primarily involves replacing the reagents used in the synthesis of UiO-MOFs, such as modifying the organic linkers to introduce various functional groups into the UiO frameworks[35]. This method enables the preparation of modified UiO materials with high purity and uniform quality. It also provides considerable flexibility and controllability. By adjusting reaction parameters such as temperature, pressure, and solvent, the crystal shape, morphology, and pore structure of the resulting UiOs can be effectively tailored to meet specific application requirements. However, this approach can be challenging due to the unpredictability associated with introducing functional species, particularly those with large molecular sizes, into the UiO synthesis system.
PSM is also an effective approach to introduce functional groups into UiO-MOFs by modifying metal clusters or ligands after the initial synthesis. PSM enables the incorporation of a variety of functional moieties into the framework while preserving the original UiO structure. Through this method, UiO materials can be functionalized with various auxiliary groups and/or combined with other materials to form composites. The in situ growth method[36,37] is commonly employed to generate UiO-based composite materials. Other approaches, such as electrostatic self-assembly[38], microwave-assisted synthesis[39], and ultrasonic mixing[40], are also utilized for preparing UiO-containing composites. Compared with de novo synthesis, PSM allows for the introduction of a broader range of functional species, including those that are sensitive to or incompatible with the conditions required for constructing UiO frameworks, as well as those that might otherwise interact with undesirable sites and produce unwanted byproducts. This makes PSM a highly suitable method for tailoring and optimizing modified UiOs and their composites for specific applications. However, the distribution of functional units in materials prepared by PSM is often less uniform than that achieved through de novo synthesis.
3. Research Progress of UiO-MOFs in Photocatalytic CO2RR
Photocatalytic CO2RR technology enables the light-driven catalytic conversion of CO2 into sustainable, renewable clean fuels or high value-added chemicals. Depending on the reaction pathway, different reduction products can be obtained, mainly including CO, CH3OH, HCOOH, HCHO, and CH4. In a photoactive MOF material, the highest occupied molecular orbital (HOMO) level is primarily determined by the HOMO of the organic ligand, while the lowest unoccupied molecular orbital (LUMO) level is mainly influenced by the redox potential of the metal-oxygen cluster. As a result, oxidation and reduction reactions generally take place on the organic linker and the metal-oxygen cluster, respectively.
The LUMO and HOMO energy levels of MOFs can be precisely tuned within a narrow range. For an MOF photocatalyst to be active in CO2 reduction, its LUMO must be at a higher energy level than the redox potential (E0) of the corresponding CO2 reduction half-reaction, which varies depending on the target product. For example, the E0 for CO2 reduction to CO is -0.53 V (vs. NHE at pH 7), while the values for reduction to HCOOH, CH3OH and CH4 are -0.61 V, -0.38 V and -0.24 V, respectively (Figure 2). The high degree of compositional and structural tunability of MOFs makes it possible to design photocatalysts with suitable LUMO levels to drive CO2 reduction toward specific desired products.
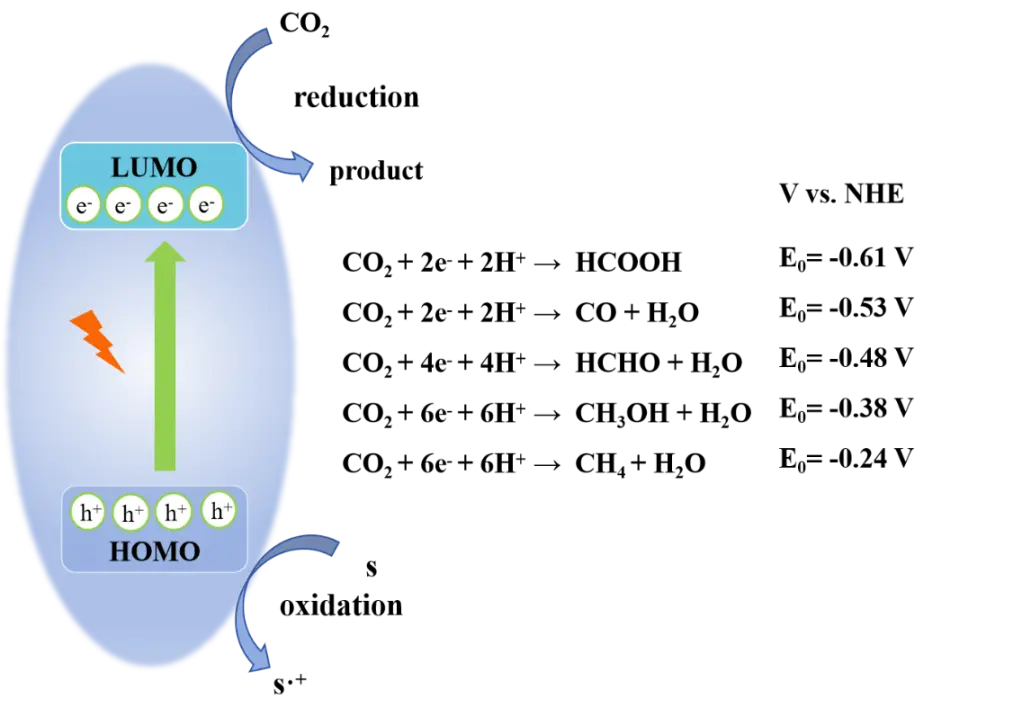
Figure 2. Photocatalytic CO2 reduction over MOFs. LUMO: lowest unoccupied molecular orbital; HOMO: highest occupied molecular orbital; MOFs: metal-organic frameworks.
For most reported UiO-based photocatalysts, the primary products of photocatalytic CO2RR are CO and HCOOH. This is mainly because the LUMO energy levels of most UiO-derived catalysts are more negative than the redox potentials (E0) required for the reduction of CO2 to these products. In addition, the complexity of the reaction pathways influences product selectivity. The conversion of CO2 to CO or HCOOH involves a relatively simple two-electron transfer process, which is easier to achieve. In contrast, the formation of other single-carbon or C2+ requires multi-electron transfer and/or multi-step reactions, making these transformations more challenging.
Pure UiO materials exhibit poor performance in photocatalytic CO2RR, and the main reasons are as follows: (1) pure UiOs have relatively broad band gaps and almost no visible light absorption, which limits their light utilization ability; (2) the relatively long electron transport path in pure UiO materials makes it easy for photoproduced carrier recombination; (3) pure UiO materials possess low chemical adsorption capacity for CO2 and lack a stabilizing effect on key intermediates of CO2RR; (4) the single surface active site of pure UiO materials sometimes cannot meet the demand for multi-step reactions and drive multi-electron transfer processes. Thus, to enhance the photocatalytic performance of UiO materials for CO2RR, several modification methods have been developed, including (i) band-gap engineering by regulating the metal node or organic linker to adjust the position of the energy band level, (ii) defect engineering to introduce impurity energy levels and modulate the local electronic structure, (iii) introduction of metal single atoms (SAs) or nanoparticles (NPs) to boost charge separation efficiency, and (iv) combination with other photoactive semiconductors to construct interfacial built-in electric fields for facilitating the separation and migration of photoproduced carriers. These modification strategies will be discussed successively in the subsequent sections of this article.
3.1 Band-gap engineering
The photocatalytic activity of UiOs can be improved by regulating their band structures through metal node modulation or organic ligand functionalization (Figure 3). For photocatalytic CO2RR, most studies have focused on reducing the band gaps and extending the visible-light photoresponse ranges of UiO-based materials to enhance their photocatalytic efficiency.
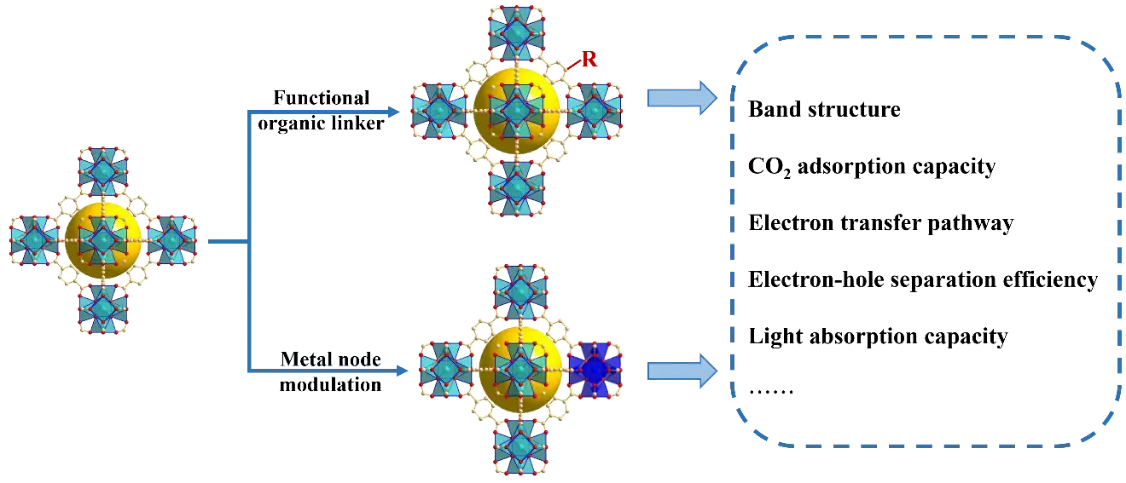
Figure 3. Schematic diagram of two typical types of band-gap engineering of UiO-66.
3.1.1 Modulation of organic linker
Modifying the bridging linker is an effective approach to alter the energy band level positions of UiO-MOFs. The introduction of ligands with different functional groups can change the HOMO levels of UiO materials, thereby adjusting their band gap widths and physicochemical properties. For example, the presence of -CH3 and -NH2 groups on H2BDC can reduce the band gap of UiO-66 to varying degrees due to their differing electron-donating abilities, which lead to different extents of upward shifts in the HOMO level[41]. Specifically, substitution -NH2 and -CH3 groups can reduce the band gap of hcp UiO-66 (originally 4.07 eV) to 2.92 eV and 3.93 eV, respectively. In contrast, -COOH functionalization increases the band gap[42], limiting the optical response of the material to the ultraviolet region. As shown in Figure 4, a variety of linkers have been employed in the syntheses of UiO derivatives.
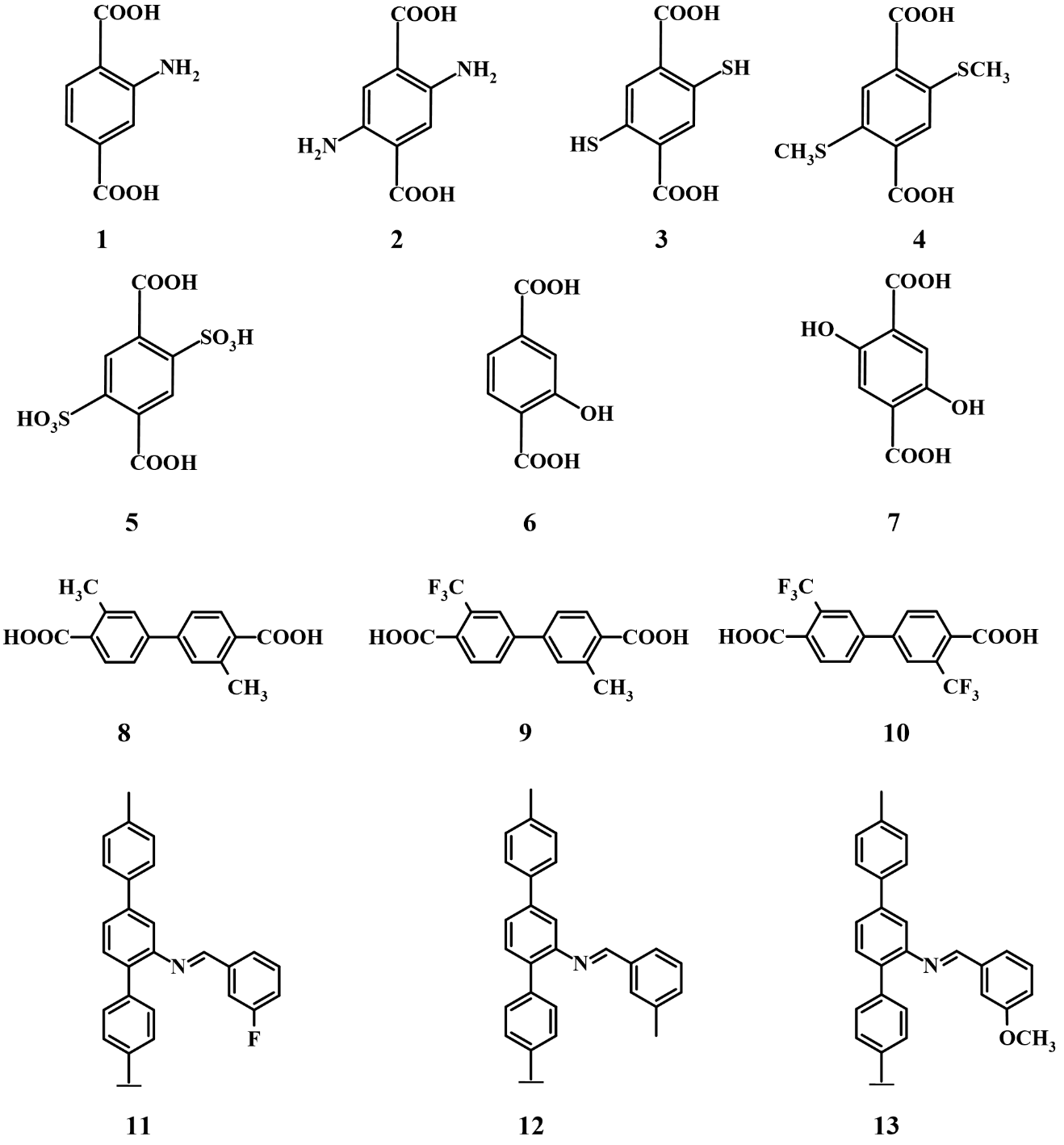
Figure 4. Different organic linkers used to synthesize UiO-MOFs or modified after synthesis. MOFs: metal-organic frameworks.
Light-harvesting modification of UiOs began with the work of Silva et al.[43], which demonstrated that the presence of -NH2 groups on the linker in UiO-66 extended its absorption spectrum into the visible light region, thereby enhancing its photocatalytic performance for the hydrogen evolution reaction. In addition, it has been well established that the light-harvesting capability can be further improved by increasing the number of functional groups in the ligand or by using mixed linkers. Sun et al.[44] synthesized a mixture of NH2-UiO-66 and 2,5-NH2-UiO-66 using organic linkers containing one and two -NH2 groups (1 and 2), which exhibited showed higher photocatalytic CO2 reduction activity toward HCOOH (41.4 μmol·g-1·h-1) compared to pure NH2-UiO-66. This improvement was mainly attributed to the enhanced light absorption and CO2 adsorption resulting from the presence of additional -NH2 groups.
UiO-66-X2 (X = -SH, -SCH3, -SO3H) synthesized by modifying H2BDC with different sulfur-containing groups (compounds 3 to 5), exhibited significantly increased band edge wavelengths in the range of 425~470 nm compared with pure UiO-66 (320 nm). This enhancement was found to depend on the electron-donating ability, geometry, and size of the functional group[45]. Among these, -SCH3 was the most effective, and UiO-66-(SCH3)2 showed a markedly reduced band gap (2.80 eV vs. 4.07 eV for UiO-66) along with improved photocatalytic performance. In addition, it has been demonstrated that introducing -OH and double -OH groups (compounds 6 and 7) into UiO-66 can also reduce its band gap to 2.2 eV[46]. Wang et al.[47] prepared three functionalized UiO-67 materials using different ligands (compounds 8 to 10). Without the use of any organic solvents or sacrificial agents, UiO-67-o-(CH3)2 exhibited an excellent CO yield for photocatalytic CO2RR, reaching 178 μmol·g-1·h-1. This enhancement can be attributed to the fact that, compared with the -CF3 group, the -CH3 group increases CO2 adsorption capacity and reduction potential while facilitating the separation and transfer of photogenerated charge carriers.
In addition to the direct synthesis of UiO-MOFs using functional bridging ligands, a PSM approach can also be employed to introduce functional species onto the linkers of UiO materials. Three UiO-68 MOFs with F-, CH3- and OCH3- functionalized linkers (compounds 11 to 13) were prepared via amine-aldehyde condensation of UiO-68-NH2 with 3-fluorobenzaldehyde, 3-methylbenzaldehyde, and 3-methoxybenzaldehyde, respectively[48]. These materials showed varying photocatalytic performance for CO2RR toward CO due to differences in charge separation and band gap characteristics. Among them, UiO-68-OCH3, containing an electron-donating group, exhibited the best catalytic ability, achieving a CO production rate of 118.12 μmol·g-1·h-1 under visible light irradiation.
In short, introducing functional groups into the organic ligands of UiOs influences their charge separation efficiency, light-harvesting ability, and electron density around the metal nodes. In particular, increasing the electron density through electron-donating groups can reduce the band gap energy of UiO materials and enhance their absorption in the visible light range, thereby significantly improving their photocatalytic performance. However, the enhancement effect of functional group introduction on linker-to-metal charge-transfer (LMCT) excitation is limited, and the variety of applicable substituents remains restricted.
3.1.2 Modulation of metal nodes
Modulation of metal centers is another effective strategy to alter the energy band level positions of UiO materials. Constructing mixed-metal nodes in UiO-MOFs through metal substitution can introduce intermetallic charge transfer and enhance optical properties, thereby improving photocatalytic performance. By partially or fully replacing Zr metal nodes with other metals such as Ti[49-51], Ce[52], Cu[53], and Hf[54], electron transfer between the different metal centers can be facilitated. This modification lowers the E0 of the metal center, narrow the effective band gap of UiO materials, and enhances charge separation efficiency[49].
Sun et al.[50] prepared a Ti-substituted UiO material, NH2-UiO-66(Zr/Ti), via a post-synthetic exchange (PSE) treatment. This material exhibited enhanced photocatalytic activity for CO2RR toward HCOOH, achieving a yield of 580 μmol·mol-1·h-1 under visible light (420 ~ 800 nm), compared with 340 μmol·mol-1·h-1 for the unsubstituted analogue. Electron spin resonance characterization and density functional theory (DFT) calculations confirmed that Ti atoms contributed significantly to the bottom of the conduction band (CB) in the modified catalyst, promoting Ti-mediated electron migration from the 2-aminoterephthalic acid linker to the Zr center, thereby improving catalytic performance (Figure 5a). Subsequently, a mixed-ligand and mixed-metal UiO-66 material was also synthesized via the PSE method and applied as a photocatalyst for CO2RR[51]. Similarly, results indicated that the presence of the Ti moiety was essential for the visible-light-driven photocatalytic CO2RR, as it enabled the metal nodes to accept electrons from the ligands. In addition, the use of a mixed ligand system consisting of 2-amino- and 2,5-diaminoterephthalic acid further enhanced the photocatalytic performance of the UiO catalyst by introducing new energy levels, thereby broadening the optical response range and facilitating charge migration.
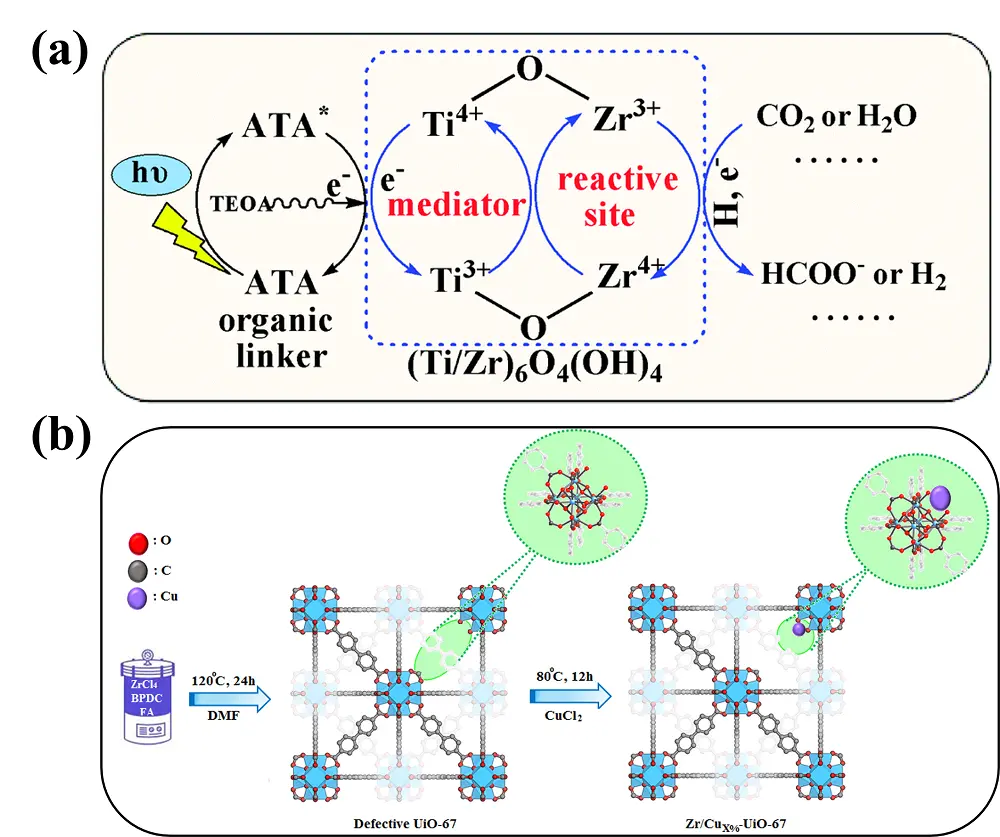
Cu has also been employed to modify the metal nodes of UiO-67. Bimetallic UiO-67-(Zr/Cu) materials were prepared by in situ anchoring of Cu atoms onto the Zr clusters of linker-defective UiO-67 (Figure 5b)[53]. Experimental results demonstrated that the formation of Zr-O-Cu sites effectively promoted CO2 adsorption, charge migration, and charge separation. Moreover, the bimetallic synergistic effect facilitated the generation of C2 intermediates during the CO2RR. As a result, the optimized catalyst with a Cu loading of 2 wt% exhibited excellent photocatalytic activity (69.1 μmol·g-1·h-1) and 100% selectivity for the conversion of CO2RR to C2H4 under visible-light irradiation.
In addition to PSE, an in situ metal exchange process was also employed to fabricate a Ti-modified NH2-UiO-66 material[49]. A decrease in band gap was observed for both catalysts synthesized via post-synthetic and in situ metal exchange methods. Despite differences in synthesis routes and Ti loadings, both catalysts exhibited significantly enhanced photocatalytic performance for CO2RR toward HCOOH production, attributed to their broadened light absorption range, which was nearly twice that of unmodified NH2-UiO-66. Beyond monometallic substitution, multimetallic UiO-66 materials incorporating Ce, Ti and Zr were also developed for the selective photocatalytic methanation of CO2 under simulated sunlight, using RuOx as co-catalyst[52]. It was found that the Zr/Ce/Ti-UiO-66 catalyst outperformed monometallic Zr- and Ce-UiO-66, as well as bimetallic Zr/Ce- and Zr/Ti-UiO-66, achieving a CH4 production rate of 1,900 μmol·g-1 after 22 hours of photoreaction. Spectroscopic characterization and photocatalytic experiments confirmed that the excellent activity was due to a dual photothermal and photochemical mechanism.
In the Zr6SBUs of UiO materials, partially or completely replacing the Zr metal nodes with other metal ions is an effective strategy to shift the band edge and band gap position, modify the hybrid energy levels between the SBU and the organic ligand, and achieve electron localization at the metal center.
3.2 Defect engineering
Defects in MOF materials are generally classified into two categories: metal defects and ligand defects (Figure 6). Metal defects typically result from the absence of metal nodes or the partial replacement of metal ions by others metal species. Ligand defects, on the other hand, often arise from the absence of organic linkers or the substitution of linkers with other molecules. It is widely accepted that the crystal structure determines the properties of materials, and the formation of structural defects is usually accompanied by bond breakage and recombination, lattice deformation, and electron localization[55]. These microstructure changes caused by defect engineering can either alter the original properties or impart new functionalities to the materials. In addition, the formation of surface defects and internal nanopores also facilitates molecular adsorption[56]. Defect engineering therefore provides an efficient strategy to regulate the physicochemical properties of MOFs, including their stability, adsorption capacity, and catalytic performance[57]. Moreover, defects can act as active sites and promote the separation of electrons and holes[58]. As such, defect engineering is considered an effective method for tuning the band structure and electronic properties, and for customizing the functionalities of MOF materials[59].
In the case of UiO series of MOFs, the strong chemical bonds between Zr and oxygen atoms, as well as the high coordination of each Zr6SBU with 12 organic linkers, make these materials highly tolerant to structural defect modulation. Defects in UiO materials generally arise from the absence of ligands and/or Zr-O clusters during the nucleation or PSM process. For UiO-66, it has been shown that the framework structure can be maintained even when each Zr-O cluster lacks up to 4.3 organic linker ligands[60]. The main strategies for introducing structural defects in UiOs include the following: (a) Replacement of Zr precursor: Using ZrOCl2·8H2O instead of ZrCl4 allows competition between the dicarboxylate organic linker and water molecules in ZrOCl2·8H2O[61], thereby reducing coordination between the Zr cluster and the ligand. (b) Solvent-free synthesis[62]: This method reduces the solubility of organic linkers, leading to coordination discrepancies between clusters and ligands. (c) Use of modulators[63,64]: Monocarboxylic acids such as HCOOH, benzoic acid, CH3COOH, and CF3COOH can act as modulators, forming defects in UiO frameworks through various mechanisms, including inducing ligand deficiency via competitive coordination, etching the lattice through chemical reactions, and regulating crystallization dynamics by altering the reaction environment. (d) Introduction of competitive metal precursors: This approach can form mixed-metal nodes and Zr6 cluster-missing defects, such as introducing six-coordinated Ti in place of some octa-coordinated Zr ions[61]. (e) PSE[65]: Through solvent-assisted ligand exchange, specific species can be anchored at defect sites generated by modulator-induced competitive coordination, enabling the synthesis of UiO materials with diverse functional groups and characteristics.
Defect structures have a significant impact on the photocatalytic performance of UiOs. Wang et al.[63] synthesized several UiO materials, denoted as UiO-6X-NH2 (X = 6, 7, and 8), containing different dicarboxylate linkers and NH 2-modified defects, and evaluated them for photocatalytic CO2RR. Among them, the 3,5-diamino-benzoate-modulated NH2-UiO-66 exhibited the highest catalytic activity for CO production (17.5 μmol·g -1·h-1), attributed to its lowest absorption energy (Eabs), achieving 2.4 times the activity of benzoate-modulated UiO-68-NH2. Subsequently, the authors prepared a series of UiO-66-NH2-X materials with defect types, including ligand vacancy defects (X = LV), missing-cluster defects (X = MC), and linker defects compensated by CH3COOH and HCOOH (X = AA and FA, respectively) (Figure 7a). Theoretical calculation and experimental results confirmed that UiO-NH2-LV exhibited the best photocatalytic CO2RR activity among the four, with a CO yield of 30.5 μmol·g-1·h-1, which was 9.2 times higher than that of UiO-66-NH2-MC (Figure 7b). DFT calculations revealed that the presence of defects would adjust the values of LMCT energy (ELMCT) and Eabs during photocatalysis, thereby resulting in different photocatalytic activities over these UiO-66 derivatives. UiO-NH2-LV possessed the smallest sum of ELMCT and Eabs, which effectively reduced the energy barrier in the rate-controlling step of CO2 reduction. Furthermore, UiO-66-NH2-LV demonstrated excellent stability and recyclability, maintaining its catalytic activity over six consecutive reaction cycles (Figure 7c).
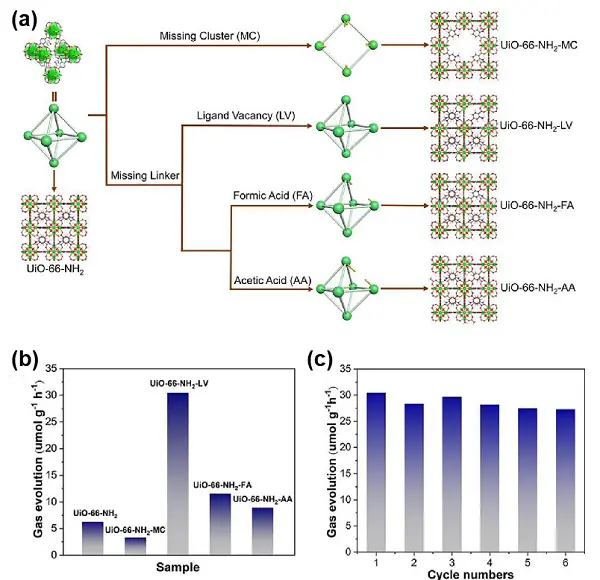
Figure 7. (a) Different defect synthesis routes of UiO-66-NH2; (b) Photocatalytic performance of various catalysts and (c) Cyclic experiments of UiO-66-NH2-LV. Republished with permission from[64].
In addition to defect type, the degree of ligand vacancies in UiOs materials can also influence their photocatalytic activity. It has been found that a greater number of ligand vacancies per metal node in UiO-66 leads to a lower ELMCT, resulting in enhanced CO evolution[66]. A series of UiO-67 materials with varying numbers of linker defects were synthesized by adjusting the amount of HCOOH used during synthesis, and they exhibited different photocatalytic CO2 reduction activities[67]. Among them, the catalyst UiO-67-200 showed the best catalytic performance for converting CO2 to CO, achieving a yield of 12.29 μmol·g-1·h-1, which was 5.25 times that of UiO-67-0. These results demonstrated that an appropriate level of linker defects can enhance the separation efficiency of charge carriers and promote LMCT.
By altering the synthesis temperature, several types of defective NH2-UiO-66 materials were prepared using HCl as a modulating agent and applied as photocatalysts for CO2RR[57]. Compared with non-defective NH2-UiO-66, these defective catalysts exhibited significantly enhanced photocatalytic activity, with a maximum HCOOH production rate of 129.8 μmol·g-1·h-1. The improved catalytic performance was attributed to the more open framework structure and the increased number of active sites generated through defect engineering, which facilitated the transfer of photogenerated charge carriers.
In summary, defect engineering, achieved by adding modulators, adjusting synthesis temperatures, and altering synthesis precursors, can create more open frameworks and increase the number of active binding sites in UiO-MOFs. As a result, it can effectively accelerate photoinduced charge transfer and suppress the recombination of photogenerated charge carriers, thereby enhancing the photocatalytic CO2 reduction capacity of UiO materials.
3.3 Introduction of metal species
In recent years, numerous studies have focused on enhancing the photocatalytic performance of UiO-MOFs by doping or loading metal species into UiOs (Figure 8). Introducing metal components enables both metal-to-metal and metal-to-cluster charge transfer, providing an effective strategy to expand the light absorption range and improve charge separation efficiency, thereby enhancing the overall photocatalytic performance of UiO catalysts.
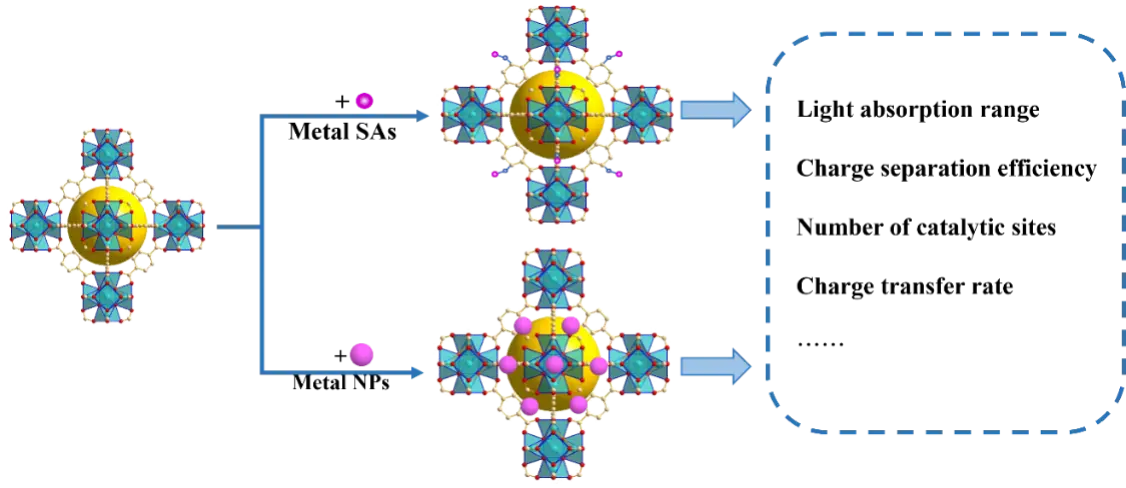
Figure 8. Schematic diagram of the modification of UiO-66 with metal species. SAs: single atoms; NPs: nanoparticles.
3.3.1 Introduction of metal single-atom site
Single-atom catalysts have attracted significant attention due to the unsaturated coordination environment and unique electronic structure of atomically dispersed active sites. These catalysts enable high atomic utilization and provide abundant photocatalytic active sites. Anchoring metal SAs onto the surface of UiO materials is considered an effective approach to address the issue of insufficient photogenerated charge separation. Moreover, the incorporation of single-atomic sites into UiOs can introduce additional photocatalytic active sites and facilitate charge transfer, thereby enhancing overall photocatalytic performance. Fe-, Co-, Ni-, and Cu-based single-atom catalysts have been widely studied for photocatalytic CO2RR, as their unfilled 3d orbitals can efficiently accelerate the transfer of photogenerated charges in the system[68]. It is important to note that these metal sites introduced into UiOs do not form part of the metal nodes. Instead, they are located on the surface or are chemically bonded to the organic linkers or Zr-oxygen clusters.
A photoinduction method was developed by Wang et al.[69] to anchor Cu SAs onto the -NH2 moieties of NH2-UiO-66 (Figure 9a). Compared with pristine NH2-UiO-66 and the Cu NPs/NH2-UiO-66 composite, the synthesized Cu SAs/NH2-UiO-66 exhibited significantly enhanced visible-light photocatalytic performance for CO2RR, producing CH3OH and C2H5OH at rates of 5.33 and 4.22 μmol·g-1·h-1, respectively. This high catalytic activity was attributed to the introduction of Cu SAs, which facilitated electron enrichment on the catalyst surface and improved photogenerated charge separation. Similarly, Zn SAs were anchored onto NH2-UiO-66, and the resulting catalyst demonstrated excellent activity for the reduction of CO2 to CO[70]. In another study, the photocatalytic performance of Bipy-UiO-67 was enhanced by incorporating Co SAs. The modified catalyst exhibited improved CO2 adsorption capacity and CO evolution efficiency for photocatalytic CO2RR compared to the pristine Bipy-UiO-67[71].
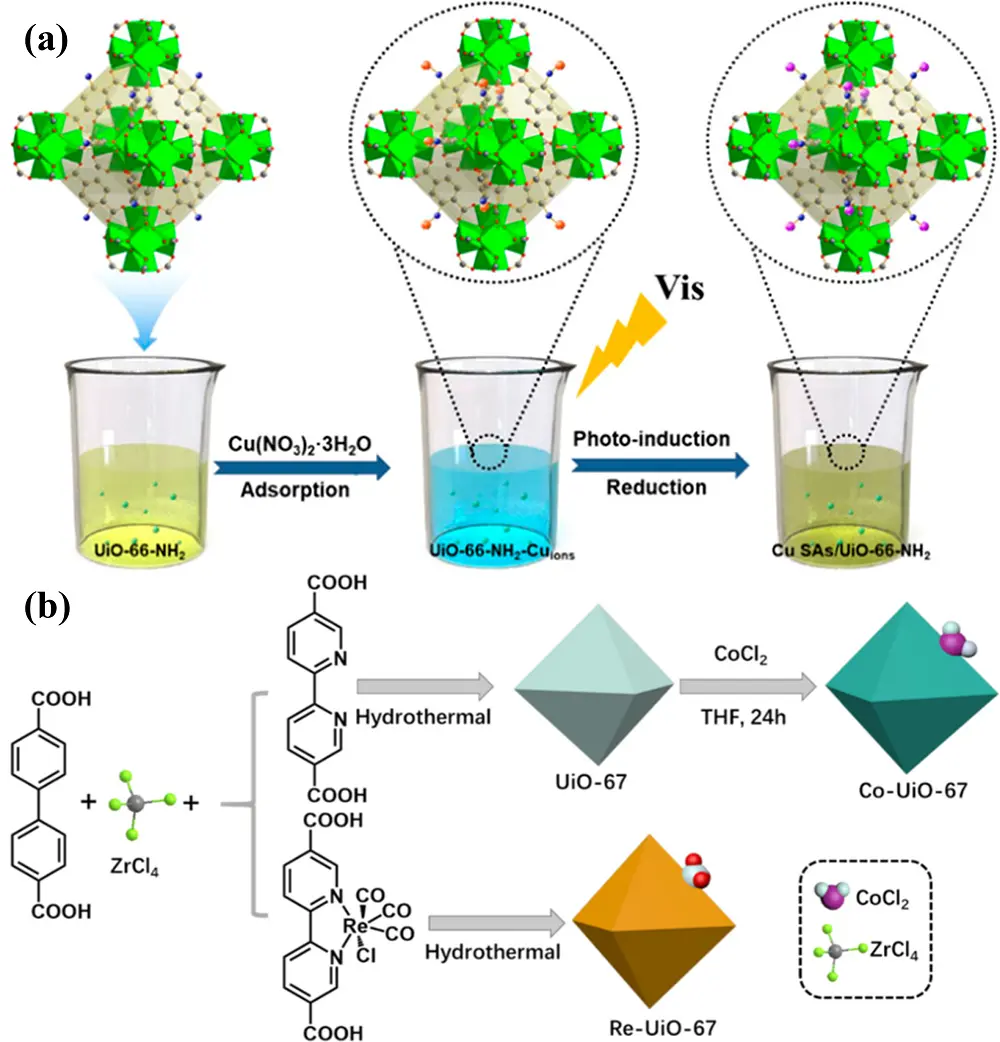
Using UiO-66-(OH)2 as the structural precursor, single-atomic Co2+ sites were anchored onto the Zr-O nodes of metal-organic-layer nanocages synthesized via a cosolvent approach[72]. The photocatalytic performance of the resulting catalyst was evaluated for CO2RR and showed an exceptionally high CO production rate of 7,740 μmol·g-1·h-1, along with excellent stability, maintaining 97.1% product retention after five cycles. This outstanding photocatalytic activity was primarily attributed to the unique nanocage structure, which facilitated greater exposure of Co2+ sites through the large-area 2D UiO-66-based nanosheets and enhanced local CO2 due to the presence of abundant surface hydroxyl groups.
In addition to metal SAs, incorporating metal complexes into UiOs is also an effective strategy to introduce isolated metal sites and enhance catalytic activity. Re(CO)3Cl-modified UiO-67[73] was synthesized using a rhenium complex bearing dicarboxylic acid functionality and applied in photocatalytic CO2RR to produce CO, achieving a high turnover number of 10.9, which was three times that of the homogeneous Re complex. Four types of half-sandwich Ru and Rh molecular complexes were incorporated into UiO-67 frameworks via a PSE strategy and used as photocatalysts for CO2RR[74]. It was demonstrated that catalysts containing Rh-OH2 or Ru-OH2 exhibited superior catalytic activity compared to those containing Rh-Cl or Ru-Cl. Two metal ion-doped UiO catalysts, Re-UiO-67 and Co-UiO-67, were synthesized through a combined solvothermal and post-metallization strategy (Figure 9b)[75]. Both UiO-67-based MOFs showed significantly higher CO2 adsorption capacities compared to the pristine material. Among them, Co-UiO-67 exhibited much higher photocatalytic activity for CO2RR with a CO evolution rate of 3,292.5 μmol·g-1·h-1, in contrast to 412.5 μmol·g-1·h-1 for Re-UiO-67. This superior performance was attributed to the enhanced charge transport properties of Co-UiO-67 and the unsaturated coordination of its Co active sites, which improved CO2 adsorption capacity.
To investigate the role of the coordination environment around single metal sites in photocatalysis, UiO-Co-Nx (x = 2, 3, 4) catalysts with different numbers of coordinated N atom numbers around Co sites were synthesized based on the UiO-67-20% material that was obtained by a mixed-ligand strategy with 20% H2bpydc and 80% H2bpdc[76]. Among these catalysts, UiO-Co-N3 exhibited the highest photocatalytic performance for CO2 conversion to CO. This superior activity was attributed to its fast charge transfer dynamics and the low electronic density of Co(II) sites, which resulted in reduced energy barriers for CO2RR.
The unique structural tunability of UiOs makes them an ideal platform for the introduction of catalytic single metal sites and the precise modulation of their coordination microenvironment, thereby enabling excellent photocatalytic performance for CO2 reduction.
3.3.2 Introduction of metal NPs
In photocatalytic reactions, metal NPs introduced into UiOs can not only enhance absorption in the visible-light spectrum but also serve as electron acceptors and active sites. Certain metal NPs, such as Au, Ag, and Cu, can broaden the optical response range through localized surface plasmon resonance (LSPR) when confined with nanoscale UiO particles. In particular, the collective oscillation of electrons on the surface of these metals, when restricted within a nanoscale cage, may give rise to characteristic optical response bands.
The Au/NH2-UiO-66/graphene (GR) catalyst was synthesized by co-modifying NH2-UiO-66 with Au NPs and GR[77]. Although the Au particles exhibited a wide size distribution (3 ~ 33 nm), the catalyst still demonstrated higher photocatalytic CO2 reduction activity and greater selectivity toward HCOOH compared to NH2-UiO-66/GR and pristine NH2-UiO-66. This improvement was attributed to the LSPR effect of the Au NPs, which enhanced visible light absorption, and the excellent conductivity of GR sheets, which helped suppress the recombination of photogenerated electron-hole pairs.
In addition, the spatial position of precious metal NPs relative to UiOs has a significant impact on photocatalytic efficiency. Two modified NH2-UiO-66 materials, with Pt NPs located on the surface and inside the nanochannels, namely Pt/NH2-UiO-66 and Pt@NH2-UiO-66 respectively, both exhibited superior photocatalytic performance compared to pristine NH2-UiO-66[78]. Notably, Pt@NH2-UiO-66 showed approximately five times the efficiency of Pt/NH2-UiO-66, primarily due to its shortened electron migration distance, which facilitated the separation of photogenerated electron and hole pairs. Similarly, a series of hybrids incorporating Pt NPs (approximately 4 nm in size) in different positions, interior, exterior, or both interior and exterior, of flower-like NH2-UiO-68 were synthesized using various preparation methods and Pt loadings (Figure 10)[79]. Among them, Pt(2)@NH2-UiO-68, with 2 wt% Pt loaded inside the porous framework, exhibited the highest visible-light photocatalytic activity for the conversion of CO2 to CO. These results demonstrated that proper positioning and loading of Pt NPs within the catalyst can enhance their interaction with NH2-UiO-68 and facilitate charge transfer during the photocatalytic process.
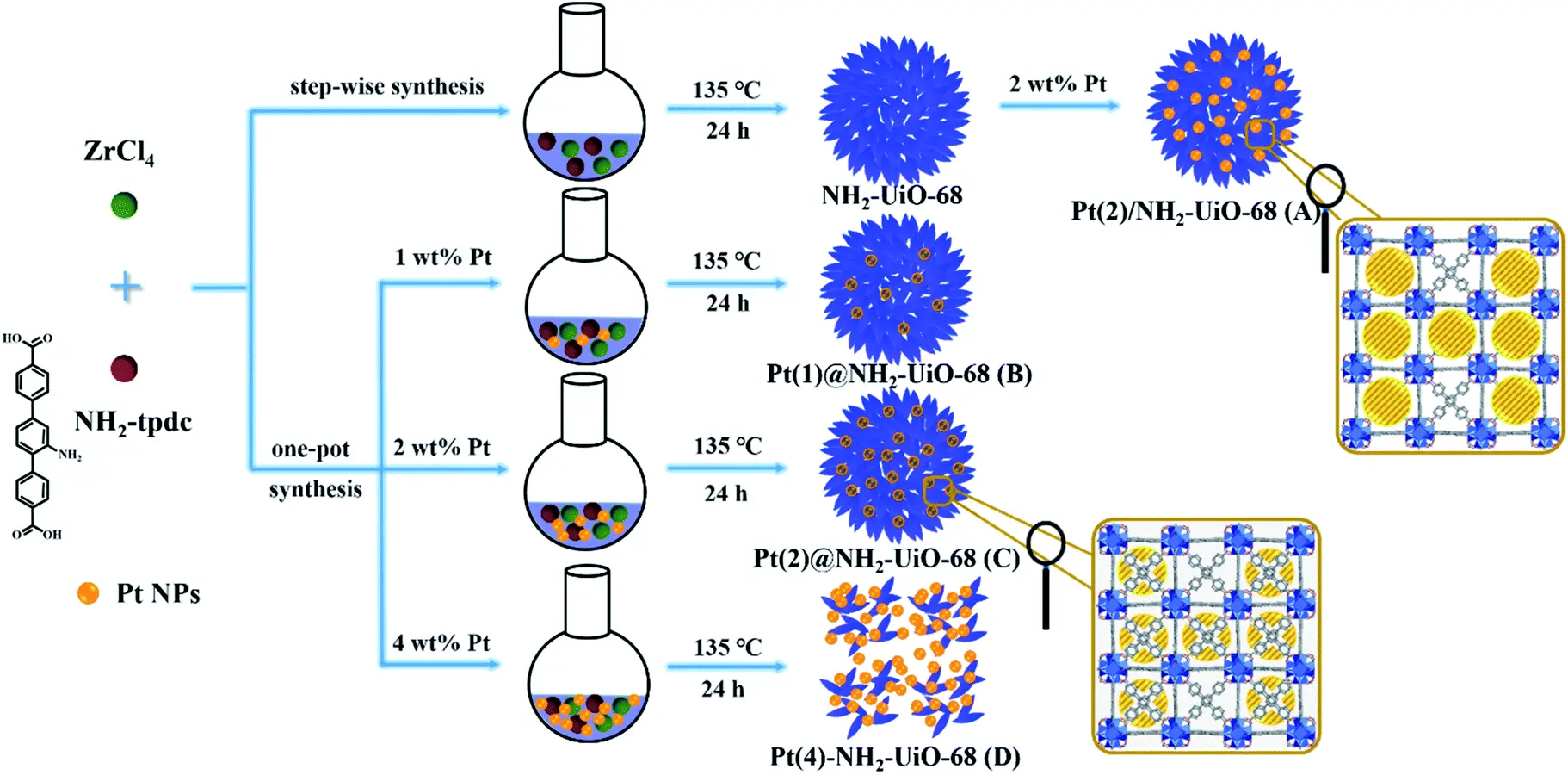
Figure 10. Synthesis procedures of various Pt NP-loaded UiO-68 catalysts. Republished with permission from[79]. NPs: nanoparticles.
Furthermore, the size of metal NPs also has a significant impact on catalytic efficiency. Four Ag@UiO-66 catalysts with varying sizes and loading of Ag NPs were prepared via in situ growth of Ag NPs within the nanopores of SH-functionalized UiO-66[80]. The Ag loadings on these catalysts were 8.32%, 23.27%, 39.79%, and 51.20%, with corresponding Ag NP sizes of 1.06 nm, 1.9 nm, 2.0 nm, and 5.0 nm, respectively. Photocatalytic tests showed that the catalytic activities of Ag@UiO-66 followed a volcano-type trend as the size of Ag NPs increased. The catalyst with an average Ag particle size of 2.0 nm and a loading of 39.79% exhibited the best activity for photocatalytic CO2RR to produce CO as well as a small amount of hydrocarbons, due mainly to its good balance in Ag loading and mass transfer within the nanochannels.
Beyond noble metals, non-noble metal NPs have also been introduced into UiOs for photocatalytic CO2RR. Several Co-decorated NH2-UiO-66 catalysts with varying Co loadings were synthesized by post-synthetic NaBH4 treatment[81]. The catalyst with 5wt% Co loading showed the most prominent photocatalytic activity for CO2RR, with a CO evolution rate of 1,930 μmol·g-1·h-1, which was 10.2 times that of the unmodified catalyst. This high catalytic performance was mainly due to the high dispersion of Co on the catalyst surface, which accelerated charge transfer and separation during catalysis. Six types of metal NPs, namely Au, Cu, Ag, Pt, Pd, and Ni, were supported onto UiO-66 as photocatalysts for CO2RR[82]. The integration of Au and Cu NPs into UiO-66 obviously boosted the catalytic performance of CO2 reduction to CH3OH and C2H5OH. It was because of the incorporation of metal NPs that leads to the LSPR, thus promoting the light absorption capacity, efficient charge dynamics, charge separation efficiency, as well as the production of electron-hole pairs.
As it turns out, introducing metal NPs into UiO-MOFs can enhance their photocatalytic performance by improving visible light absorption, promoting charge transfer during photocatalysis, or inhibiting the recombination of electron-hole pairs. However, the types of metal NPs introduced into UiO materials remain limited, and most of them are precious metal NPs. Therefore, it is necessary to continue developing more suitable materials to serve as co-catalysts.
3.4 Combination with other materials
Due to the high carrier transport resistance, low structure order, and narrow band gap of UiO materials, the separation efficiency, transfer efficiency, and adsorption capacity of photogenerated charges in pristine UiOs are far from ideal. As a result, extensive research has focused on enhancing the photocatalytic performance of UiOs by combining them with other materials, such as sulphides[37,83], lead-free halides[84-86], and other MOFs[87]. Compared with single-component catalysts, constructing composite photocatalysts offers several potential advantages. First, the introduced photoactive semiconductor can serve as a light-harvesting center to improve solar energy utilization. Second, the UiO component, with its high porosity and SSA, can provide active sites and prevent the aggregation of external NPs. Third, the formation of tight interfacial connections between the photoactive semiconductor and UiO can create effective pathways for the migration and separation of photogenerated charges.
3.4.1 As co-catalysts for photocatalytic CO2RR
In some studies, UiOs have been combined with other photocatalysts and used as co-catalysts. The primary functions of UiOs as co-catalysts in photocatalysis include providing a robust scaffold to support and stabilize other photocatalysts, as well as offering a high SSA for the adsorption of reactants. For example, a composite photocatalyst, Ni@Ru-UiO-67, with visible-light response was fabricated by encapsulating a Ni(II) molecular catalyst into Ru-UiO-67 via a “ship-in-a-bottle” strategy[88]. This composite exhibited excellent catalytic activity, selectivity, and durability for photocatalytic CO2RR toward CO. The high performance was attributed to the efficient transfer of photoelectrons generated by the Ru centers to the Ni(II) active sites. Tan et al.[89] synthesized a soluble UiO-66(NH4OH) and applied it as a co-catalyst for photocatalytic CO2RR to CO. The resulting CO yield reached 30.6 μmol·h-1 with 5.0 mg sample, which was 1.5 times higher than that of its insoluble analogue.
3.4.2 Construction of heterojunctions
Constructing heterojunctions has proven to be a highly promising strategy for modifying UiOs to enhance their photocatalytic properties, owing to its simplicity, feasibility, and high efficiency in facilitating the separation of photogenerated electron-hole pairs. Extensive research has been devoted to combining UiO-MOFs with other materials through various methods to construct heterojunctions, and significant progress has been made in this area[37,90]. Based on current studies on UiO heterojunction modification, three types of heterojunctions have been developed: type-II, Z-scheme, and S-scheme.
A type-II heterojunction consists of two staggered semiconductors (PS-I and PS-II). As shown in Figure 11a, PS-II possesses a lower oxidation potential and a higher reduction potential compared to PS-I. Under appropriate light excitation, photogenerated electron-hole pairs are produced under suitable light excitation. Electrons transfer from the CB of PS-II to the CB of PS-I, while holes move in the opposite direction, resulting in the effective separation of photoinduced electron-hole pairs. Table 1 summarizes relevant studies on UiO-based type-II heterojunction materials for photocatalytic CO2RR conducted over the past five years.
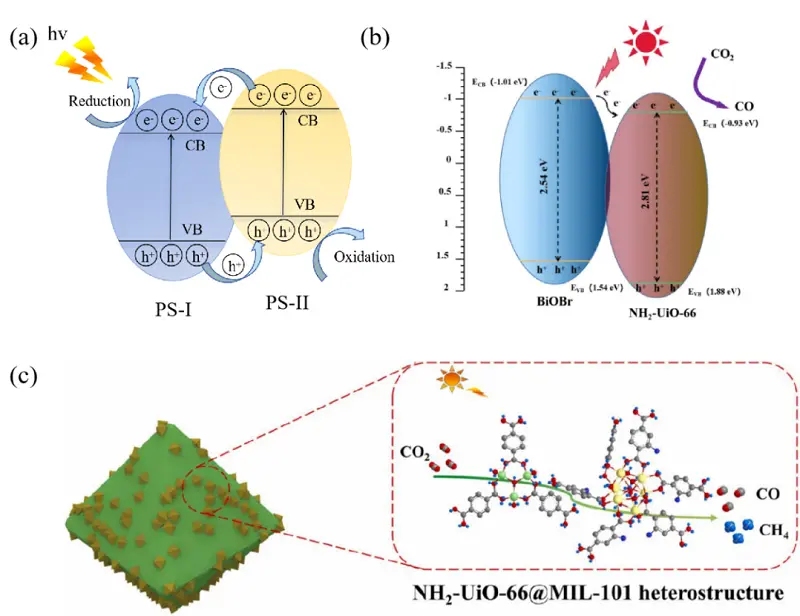
Catalyst | Conditiona | Product | Yield (μmol·g-1·h-1) | Ref. |
NH2-UiO-66/CdIn2S4 | 50 mg sample, 100 mL H2O, 25 °C, 300 W Xe lamp | CO | 11.24 | [37] |
UiO-66/Bi4O5Br2 | 30 mg sample, 50 mL H2O, 5 °C, 300 W Xe lamp | CO | 8.35 | [38] |
Cs2AgBiBr6QDs/Ce-UiO-66-H | 15 mg sample, 50 μL H2O, 300 W Xe lamp | CO | 309.01 | [84] |
BiOBr/NH2-UiO-66 | 10 mg sample, 10 mL H2O, 300 W Xe lamp | CO | 9.19 | [85] |
NH2-UiO-66@MIL-101 | 30 mg sample, 15 mL H2O, 300 W Xe lamp | CO CH4 | 267.90 18.63 | [87] |
CsPbBr3QDs/UiO-66(NH2) | 10 mg sample, H2O/ethyl acetate (1/300), 5 °C, 300 W Xe lamp, λ ≥ 420 nm | CO CH4 | 98.57 3.08 | [91] |
CuSAs@PCN@UiO | 5 mg sample, 3 mL TEA, 7 mL MeCN/H2O(6/1), λ ≥ 420 nm | CH3OH | 4,150 | [92] |
MIL-125to@UiO-66 | 1 mg sample, 0.5 mL H2O, 0.2 MPa, 300 W Xe lamp, λ > 420 nm | CO | 56.4 | [93] |
NH2-UiO-66/g-C3N4/CdTe | 2 mg sample, 4 mL MeCN, 1 mL EtOH, 300 W Xe lamp, λ > 400 nm | HCOOH | 24.6 | [94] |
g-C3N4/UiO-66(Zr/Ce) | 20 mg sample, 15 mL MeCN, 5 mL H2O, 300 W Xe lamp, λ ≥ 420 nm | CH3OH C2H5OH | 54.71 38.10 | [95] |
NH2-UiO-66/Ce(HCOO)3 | 10 mg sample, 20 mL H2O, 1 mL TEOA, 25 °C, 300 W Xe lamp | CH4 | 128.81 | [96] |
Cu2O@Cu@NH2-UiO-66 | 3 mg sample, 2 mL TEOA, 300 W Xe lamp, λ ≥ 400 nm | CO CH4 | 20.90 8.30 | [97] |
HTiNbO5/UiO-66 | 10 mg sample, 0.8 mL TEOA, 1.6 mL H2O, 300 W Xe lamp | CO | 4.10 | [98] |
N-CNDs/NH2-UiO-66 | 2 mg sample, 5 mL MeCN/TEOA(4:1), 300 W Xe lamp, λ > 400 nm | HCOOH | 80.5 | [99] |
BiOBr/UiO-66(Ce) | 10 mg sample, 10 mL H2O, 300 W Xe lamp, λ > 420 nm | CO | 20.4 | [100] |
a TEA: trimethylamine; TEOA: triethanolamine.
Some quantum dot (QD) materials, such as Cs2AgBiBr6[86] and CsPbBr3[91], have been combine with UiO-66 and its derivatives to construct type-II heterojunction photocatalysts. These composites exhibited significantly enhanced photocatalytic efficiency for CO2 reduction, primarily yielding CO, due to rapid charge separation and transfer at the interface. In particular, the Cs2AgBiBr6/Ce-UiO-66-H composite achieved a high CO generation rate of 309.01 μmol·g-1·h-1, which was approximately 2.7 and 2.1 times greater than those of pristine Ce-UiO-66-H and Cs2AgBiBr6, respectively[86]. This outstanding catalytic activity can be attributed to the following factors: (i) the close interfacial contact inhibited the agglomeration and decomposition of Cs2AgBiBr6QDs; (ii) more structural defects were introduced into the Ce-UiO-66-H framework by Cs2AgBiBr6; and (iii) good band alignment and high electron mobility promoted efficient charge separation. It can be seen that the combination of halide QDs and UiO-MOFs is conducive to the dispersion of QDs, and the constructed compact heterojunction contact surface improves electron mobility and carrier separation efficiency, thus achieving better performance in photocatalytic CO2RR.
To improve the electron-hole separation ability of BiOBr, type-II heterojunctions BiOBr/NH2-UiO-66 containing Lewis basic moieties were constructed by depositing NH2-UiO-66 onto BiOBr (Figure 11b)[85]. These composites exhibited high CO yields up to 9.19 μmol·g-1·h-1 in photocatalytic CO2RR, which was four times that of pure BiOBr. Liu et al.[92] synthesized a composite, Cu SAs@PCN@UiO, by confining polymeric carbon nitride (PCN) with Cu SAs inside defective NH2-UiO-66 nanocrystals using a straightforward low-temperature thermolysis method. Cu SAs@PCN@UiO exhibited high activity for photocatalytic CO2 conversion to CH3OH, with a remarkably high product yield of 4,150 μmol·g-1·h-1, along with small amounts of CH3CH2OH and trace CH4. It was demonstrated that both the doping of Cu SAs and the formation of heterojunction between the UiO shell and the incapsulated Cu SAs@PCN contributed to the highly efficient generation of CH3OH.
Some double MOF-based type-II heterojunctions have been developed. A type-II heterojunction material, NH2-UiO-66@MIL-101, was fabricated via a solvothermal approach by depositing nanoscale NH2-UiO-66 onto MIL-101[87]. The resulting heterostructure enhanced the separation of photogenerated charges and facilitated interfacial electron migration (Figure 11c). As a result, the composite exhibited superior photocatalytic activity for CO2RR compared with both pristine MOF components, achieving CO and CH4 production rates of 267.90 and 18.63 μmol·g-1·h-1, respectively. These rates were more than three times those of MIL-101(Cr) and approximately 5.75 times those of NH2-UiO-66. Three types of core-shell heterostructures were constructed by loading UiO-66 onto MIL-125 with different exposed crystal planes via an epitaxial growth strategy[93]. Among them, MIL-125to@UiO-66 exposing {111} crystal plane showed the highest CO formation rate, reaching 56.4 μmol·g-1·h-1, along with excellent selectivity (99%) for visible-light photocatalytic CO2RR without the need for a photosensitizer or sacrificial reagent. These values were 11.3 and 1.4 times those of pure UiO-66 and MIL-125to, respectively. This outstanding catalytic performance was attributed to the improved separation of photoproduced carrier separation via the type-II heterostructure and the exposure of more active Ti sites on MIL-125to@UiO-66.
Some studies have combined UiO-MOFs with g-C3N4 for catalyst modification to reduce quenching of photoproduced charge carriers and improve sunlight utilization efficiency[94]. A 2D g-C3N4/UiO-66(Zr/Ce) composite with a type-II heterostructure was synthesized through a solvothermal method, using g-C3N4 supported Zr and Ce SAs as metal sources of UiO-66 nanosheets, forming interfacial N-Zr/Ce-O bonds[95]. Under visible light, this composite efficiently catalyzed CO2RR to produce CH3OH and C2H5OH, with evolution rates of 54.71 and 38.10 μmol·g-1·h-1, respectively. This high catalytic activity was attributed to the facilitated delocalization and migration of photoelectrons from the conjugated π structure of g-C3N4 to UiO-66 via the N-Zr/Ce-O bonds. Additionally, the strong coordination interaction between the two materials endowed the composite photocatalyst with excellent stability during photocatalytic CO2RR, maintaining catalytic activity after 12 cycles.
Although type-II heterojunctions can to some extent enhance the separation efficiency of photogenerated electron-hole pairs, they often sacrifice the high redox capability of photocatalysts, resulting in unsatisfactory photocatalytic performance. To address this challenge, Z-scheme heterojunctions have been introduced. Under light irradiation, as illustrated in Figure 12a, electrons in the valence band (VB) in PS-I are first excited to its CB, and then migrate to the VB of PS-II. Consequently, photoproduced electrons and holes accumulate in PS-II and PS-I, respectively, which possess higher reduction and oxidation potentials. This process not only promotes effective separation of photoproduced electron-hole pairs but also optimizes the redox potential. Relevant studies on UiO-based Z-scheme heterostructure materials for photocatalytic CO2RR over the past five years are summarized in Table 2.
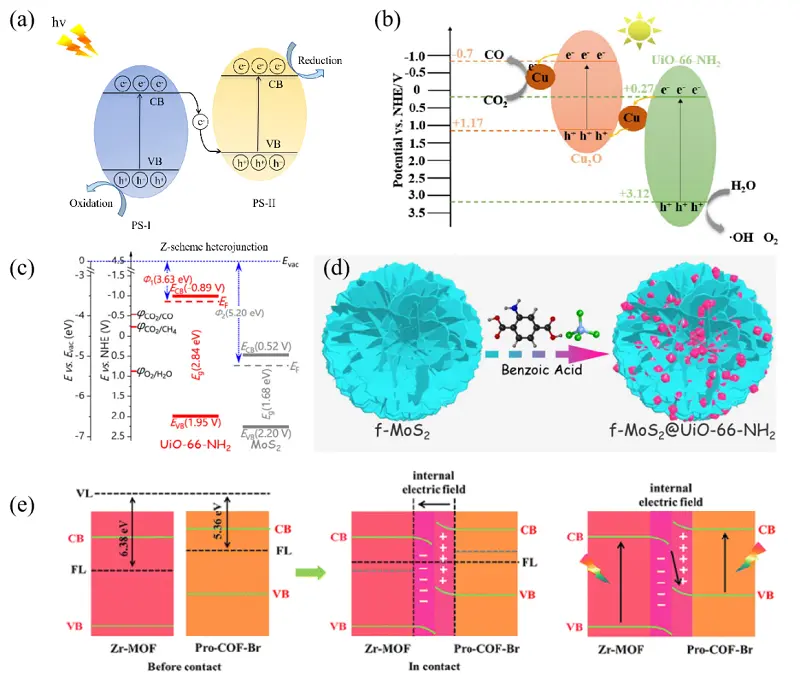
Figure 12. (a) Schematic diagram of Z-scheme heterojunction; (b) Illustration of the photocatalytic CO2RR over NH2-UiO-66/Cu2O/Cu. Republished with permission from[101]; (c) Energy level structures of NH2-UiO-66 and MoS, and (d) depiction of the synthesis of f-MoS2@NH2-UiO-66. Republished with permission from[102]; (e) Schematic diagram of electron migration in the Z-scheme heterojunction NH2-UiO-66@Pro-COF-Br. Republished with permission from[103]. CO2RR: CO2 reduction reaction; CB: conduction band; VB: valence band.
Catalyst | Condition | Product | Yield (μmol·g-1·h-1) | Ref. |
CdS@NH2-UiO-66 | 1 mg sample, 150 μL H2O, LED lamp | CO | 280.5 | [83] |
NH2-UiO-66/Cu2O/Cu | 50 mg sample, 100 mL H2O, 20 °C, 300 W Xe lamp | CO | 4.54 | [101] |
f-MoS2@NH2-UiO-66 | 5 mg sample, 4.9 mL MeCN, 0.1 mL H2O, 25 °C, 300 W Xe lamp | CO CH4 | 23.16 27.18 | [102] |
NH2-UiO-66@Pro-COF-Br | 10 mg sample, 0.3 mL 2M H2SO4, NaHCO3, 300 W Xe lamp | CO | 26.59 | [103] |
CdS/Ag-AgBr/Au-UiO-66(Ce) | 500 mL 0.1 M KOH/Na2SO3/Na2S, 2.0 M NaNO2, 500 W Xe lamp | CH3OH | 1,939a | [104] |
g-C3N4/NH2-UiO-66 | 10 mg sample, 20 mL H2O, 1 mL TEOA, 10 °C, 300 W Xe lamp, λ > 420 nm | CO CH4 | 21.65a 73.40a | [105] |
Zn-NH2-UiO-66/CeO2 | 10 mg sample, 20 mL H2O, 1 mL TEOA, 10 °C, 300 W Xe lamp, λ > 420 nm | COCH4 | 22.5253.18 | [106] |
SiW9Co3@NH2-UiO-67 | 5 mg sample, 5 mL MeCN/H2O/TEOA(48:1:1), 300 W Xe lamp, λ > 420 nm | CO | 153.3 | [107] |
SiW9Co3@NH2-UiO-68 | CO | 46.6 | ||
COF-366-Co/NH2-UiO-66 | 5 mg sample, 10 mL MeCN/H2O/TEOA(3:1:1), 10 mg [Ru(bpy)3]Cl2·6H2O, 150 W Xe lamp | CO | 4,092.16 | [108] |
Ag/UiO-66@g-C3N4 | 10 mg sample, 30 mL H2O, 80 °C, 300 W Xe lamp | CH3OH CO | 17.76 11.45 | [109] |
CuO/Ag/UiO-66 | 30 mg sample, 100 mL H2O, 0.3 MPa, 300 W Xe lamp | HCOOH | ~63 | [110] |
NH2-UiO-66/CeCO3OH | 10 mg sample, 20 mL H2O, 1 mL TEOA, 10 °C, 300 W Xe lamp | CO CH4 | 103a 70a | [111] |
O-ZnO/rGO/NH2-UiO-66 | 100 mg sample, 100 mL 0.1 M NaHCO3, 300 W Xe lamp, λ > 420 nm | CH3OH HCOOH | 34.83 6.41 | [112] |
a: The unit is μmol·g-1 ; CO2RR: CO2 reduction reaction.
The NH2-UiO-66/Cu2O/Cu material with an indirect Z-scheme heterostructure was constructed by coupling Cu2O with NH2-UiO-66 for photocatalytic CO2 conversion to CO[101]. This composite achieved CO production rates of up to 4.54 μmol·g-1·h-1, which were 37.8 and 50.4 times higher than those of NH2-UiO-66 and Cu2O, respectively. The superior photocatalytic performance was attributed to the effective formation of the Z-scheme heterostructure (Figure 12b), in which NH2-UiO-66 prevents Cu2O from photocorrosion and enhances CO2 adsorption capacity, while Cu acts as a mediator to promote electron transfer and provides active sites.
Several Z-scheme heterojunctions, MoS2@NH2-UiO-66, were synthesized by combining MoS2 with different morphologies and NH2-UiO-66 with varying grain sizes, and were applied for photocatalytic CO2 reduction[102]. Among them, the flower-like composite based on 100 nm NH2-UiO-66, as shown in Figure 12c,d, exhibited the best photocatalytic performance due to its well-matched micro-geometric configuration. It achieved a CH4 yield of 27.18 μmol·g-1·h-1 and a selectivity of 82.44%. This excellent catalytic activity can be attributed to the intimate heterointerface between the small-size NH2-UiO-66 and the flower-like MoS2, which possesses superior basal plane curvature. This configuration significantly increased the contact barrier and promoted interfacial charge transfer.
A type of core-shell Z-scheme heterojunctions NH2-UiO-66@Pro-COF-Br, was constructed by in situ growth method via the Schiff-base reaction[103]. In these hybrid materials, the porphyrin units on the Pro-COF-Br shell functioned as light-harvesting centers, greatly extending the light absorption range. At the same time, the formed Z-scheme heterostructure and the C=N bonds acted as bridges to significantly enhance charge migration and separation (Figure 12e). As a result, these composites exhibited excellent catalytic performance for CO2RR under visible light, achieving CO production rates up to 26.59 μmol·g-1·h-1.
In addition to single heterostructure, double Z-scheme heterojunctions have also been also constructed and applied for photocatalytic CO2RR. A catalyst with double Z-scheme heterojunctions was prepared by hand-grinding CdS/Ag-AgBr nanocrystals with Au-supported NH 2-defective UiO-66(Ce), and employed for the photocatalytic CO2RR to produce CH3OH[104]. The CH3OH yield over this catalyst reached 1,939 μmol·g-1 within 4 h, which was approximately 5 times and 4.4 times higher than those achieved by the single Z-scheme heterojunction systems of Au-supported NH2-defective UiO-66(Ce) and CdS/Ag-AgBr, respectively.
Based on the concept of the direct Z-scheme heterojunction, the S-scheme heterojunction was proposed to address charge transfer limitations in type-II and conventional Z-scheme heterojunctions. An S-scheme heterojunction is composed of a reduction photocatalyst (RP) and an oxidation photocatalyst (OP) with staggered band structure (Figure 13a)[113]. When RP comes into contact with OP, electrons from the RP migrate to the OP at the interface, resulting in the RP and OP sides becoming positively and negatively charged, respectively. Meanwhile, the interface between OP and RP forms downward or upward band bending and a built-in electric field that drives the transfer of photoproduced electrons from the CB in the OP to the VB of the RP. In addition, the band bending and Coulombic attraction between holes in the RP and electrons in the OP further promote this charge migration. In contrast, the built-in electric field, band bending, and Coulombic repulsion inhibit the migration of electrons from the CB of the RP to that of the OP, as well as the movement of holes from the VB of the OP to that of the RP. As a result, the S-scheme heterostructure enables both efficient charge separation and strong redox capacity, thereby enhancing photocatalytic performance. Table 3 summarizes recent studies on UiO-based S-scheme heterojunction materials for photocatalytic CO2RR over the past five years.
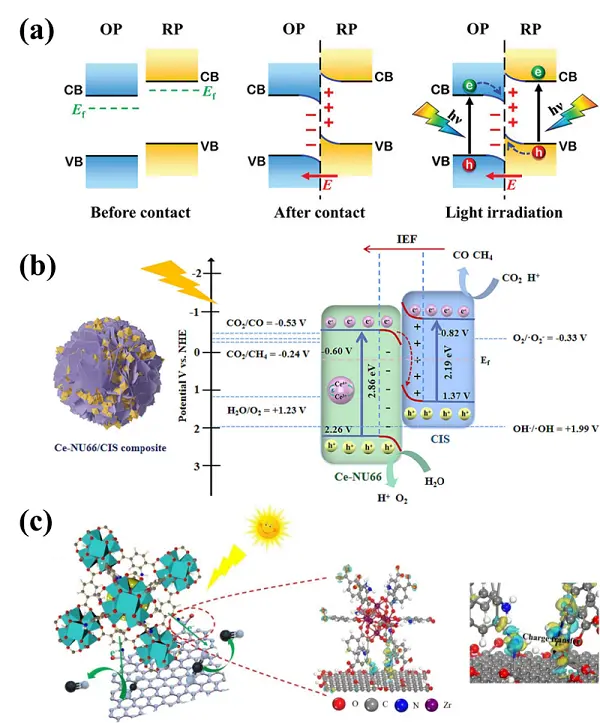
Figure 13. (a) Charge migration processes in an S-scheme heterostructure. Republished with permission from[113]; (b) Mechanism of visible light photocatalytic CO2RR over Ce/Zr-NH2-UiO-66/CdIn2S4. Republished with permission from[114]; (c) Mechanism of photocatalytic CO2RR over NH2-UiO-66/RGO and the corresponding differential charge density. Republished with permission from[115]. CO2RR: CO2 reduction reaction; CB: conduction band; VB: valence band; OP: oxidation photocatalyst; RP: reduction photocatalyst.
Catalyst | Condition | Product | Yield (μmol·g-1·h-1) | Ref. |
Ce/Zr-NH2-UiO-66/CdInS4 | H2O, simulated solar irradiation | CO | 6.01 | [114] |
NH2-UiO-66/RGO | 30 mg sample, 100 mL H2O, 20 °C, 300 W Xe lamp | CO | 23.54 | [115] |
Cs3Bi2Br9QDs@UiO-66 | 30 mg sample, 0.5 mL H2O, 80 kPa, 300 W Xe lamp | COCH4 | 41.736.17 | [116] |
NH2-UiO-66/CuZnS | 20 mg sample, 84 mg NaHCO3, 0.3 mL 2M H2SO4, 300 W Xe lamp | CO | 22.58 | [117] |
CdSeTe QDs/NH2-UiO-66 | MeCN/H2 O(4:1), TEOA, C30H24Cl2N6Ru-6H2O | CO | 228.68 | [118] |
TTCOF/NH2-UiO-66 | 5 mg sample, 5 μL H2O, 300 W Xe lamp, λ > 420 nm | CO | 6.56 | [119] |
NH2-UiO-66/SiC | 20 mg sample, 84 mg NaHCO3, 0.3 mL 2M H2SO4, 300 W Xe lamp, λ > 420 nm | CO | 7.30 | [120] |
CO2RR: CO2 reduction reaction.
A type of S-scheme heterojunction, Ce/Zr-NH2-UiO-66/CdIn2S4 with varying Ce contents, was synthesized by modifying NH2-UiO-66(Ce/Zr) with CdIn2S4 through an in situ hydrothermal co-synthesis strategy and was applied for photocatalytic CO2RR to produce CO and CH4 [114]. Without the use of any sacrificial reagents, the composite with a Ce:Zr molar ratio of 2:8 exhibited the highest CO production rate of 6.01 μmol·g-1·h-1 and a selectivity of 54.98%. These values were 3.72 and 17.83 times higher than those of CdIn2S4 and NH2-UiO-66, respectively. This high photocatalytic reduction performance was attributed to the oxygen vacancies generated by doping mixed-valence Ce ions (Ce3+/Ce4+), as well as the enhanced charge separation efficiency resulting from the formation of the heterojunction (Figure 13b).
Cs3Bi2Br9 QDs were incorporated into UiO-66 to form an S-scheme heterojunction[116], which combined the high porosity and SSA of UiO-66 with the superior power conversion capability of the QDs. It was demonstrated that the composite material Cs3Bi2Br9@UiO-66 exhibited better photocatalytic activity for CO2RR compared with the composite of large-sized Cs3Bi2Br9 and UiO-66. An S-scheme photocatalyst, NH2-UiO-66/CuZnS, was also constructed to reduce the band gap of ZnS by in situ growing NH2-UiO-66 on CuZnS via a microwave hydrothermal method[117]. This system enabled a thermally assisted photocatalytic CO2 reduction process. The reaction temperature increased to 54.2 °C due to the photothermal conversion effect of CuZnS, resulting in a CO yield of 22.85 μmol·g-1·h-1.
The covalent bonds in heterostructure composite materials can accelerate interfacial electron transfer, shorten the migration path of photoproduced electrons, and enhance the electron utilization rate. Zhao et al.[115] reported a type of covalently bonded NH2-UiO-66/RGO composite photocatalyst for CO2RR (Figure 13c). The presence of covalent bonds in these composites rendered them highly catalytically active, achieving CO yields up to 23.54 μmol·g-1, which was approximately 13 times that of pure NH2-UiO-66. Another covalently linked heterostructure, CdSeTe QDs/NH2-UiO-66, was prepared via the introduction of an acylamino group[118]. It was shown that the acylamino group functioned not only as a photogenerated charge transfer channel between the two components but also as a stabilizing agent for the QDs. The optimized catalyst achieved a CO generation rate of 228.68 μmol·g-1, which was 4 and 13 times higher than those of CdSeTe QDs and NH2-UiO-66, respectively.
The construction of heterojunction is the most effective and straightforward approach to enhance catalytic performance. It can overcome the limitations of single-component materials that suffer from inefficient electron-hole separation and can generate synergistic effects that significantly improve photocatalytic properties. Therefore, future research should focus on developing more efficient and stable heterojunction structures to further improve electron-hole separation efficiency. In addition, the actual transport pathways of electrons and holes at heterojunction interfaces require more systematic investigation.
3.4.3 Other methods
In addition to serving as a co-catalyst and forming heterojunctions, UiO-MOFs can also be combined with other materials to leverage their inherent properties for enhancing photocatalytic activity. This enhancement can be achieved by reducing the agglomeration of UiO-MOF particles, improving the conductivity or light utilization efficiency of composites, constructing strong coordination bonds, or increasing CO2 adsorption capacity.
A poly(triphenylamine) (PTPA)-decorated NH2-UiO-66 photocatalyst was prepared through a hydrothermal process[121], in which NH2-UiO-66 NPs were highly dispersed on PTPA, which possesses a large surface area (Figure 14). PTPA not only stabilized NH2-UiO-66 NPs against aggregation but also provided an electron transport channel that facilitated rapid separation of electrons and holes. The resulting composite photocatalyst exhibited enhanced CO2 adsorption capacity and improved visible light response, leading to better photocatalytic performance for CO2 reduction to HCOOH. The sample containing 30 wt% PTPA showed a photocatalytic activity 5.5 times higher than that of pure NH2-UiO-66. Using a microwave-induced approach, well-distributed UiO-66 nanocrystals were grown in situ and assembled onto GR to form an NH2-UiO-66/GR hybrid[122]. This composite displayed high activity and selectivity for visible-light-driven photocatalytic CO2RR toward HCOOH, achieving a HCOOH production rate of 443.75 μmol·g-1·h-1, which was 11 times that of pure NH2-UiO-66. The excellent catalytic performance was attributed to the small particle size of NH2-UiO-66 crystals and their uniform distribution on the GR surface, the strong CO2 adsorption capacity, and the robust interaction between the two components formed during the microwave-assisted synthesis process.
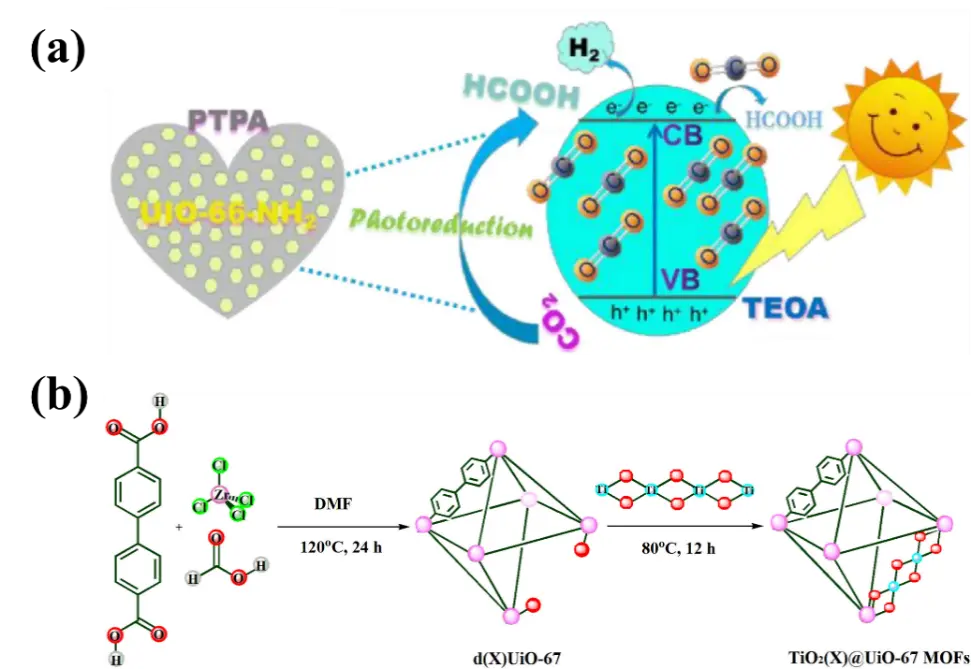
Based on UiO-67, Saadh et al.[123] developed a series of composite materials, TiO2@UiO-67, with varying Ti contents by in situ binding TiO2 nanosheets onto UiO-67 with linker defects, thereby forming charge-polarized active sites at the TiO2 and UiO-67 interface. These composites exhibited highly activity and selectivity for visible-light-driven photocatalytic CO2RR to produce ethane, achieving yields of up to 79.3 μmol·g-1·h-1 with 100% selectivity.
4. Conclusions and Prospects
Over the past few years, numerous UiO-based photocatalysts have been evolved for CO2 reduction. The significant advantages of UiO-MOFs, including large SSA, permanent porosity, abundant active sites, efficient charge separation, and high thermal and chemical stability, provide a unique platform for the development of efficient photocatalysts. As a result, related research has entered a flourishing stage. Due to the limited photocatalytic efficiency of pristine UiO materials, various modification strategies have been employed to improve their performance, mainly including band-gap engineering, defect engineering, introduction of metal species, and combination with other materials. The functional modification of UiO-MOFs and their applications in photocatalytic CO2RR have been comprehensively reviewed and discussed in this article. Through the modification of UiOs, the following primary objectives can be achieved: (1) increasing specific surface area to enhance CO2 adsorption capacity and the concentration of active sites, (2) broadening the light absorption range and improving light utilization efficiency, (3) introducing effective active sites to enhance reaction efficiency, and (4) constructing tight interfacial connections between active sites and other components to provide faster pathways for photogenerated charge carrier transfer and separation.
Although considerable advances have been made in the development of UiO-based materials for photocatalytic CO2RR, their practical applications remain limited. To address this issue, several challenges should be taken into consideration in future research.
(1) Various sacrificial electron donors are widely used in nearly all reported UiO-based photocatalyst systems; however, they have received very limited attention to date. On the one hand, it would be an interesting research direction to investigate the influence of sacrificial reagents and their reaction products on the photocatalytic performance of UiO-based materials. On the other hand, the selection of low-cost, abundant, and sustainable sacrificial agents should be carefully considered. Additionally, coupling with other photocatalytic processes such as oxidation or pollutant degradation to replace traditional sacrificial reagents is expected to enhance the overall economic feasibility.
(2) There is limited research on the photocatalytic mechanism of CO2RR over UiO-based materials. Gaining more direct evidence on the transfer process of photoproduced charge carriers would represent a significant advancement in understanding the photocatalytic mechanism. Therefore, it is highly desirable to employ in situ characterization and time-resolved detection techniques combined with DFT calculations to obtain more intuitive and accurate results by investigating the electronic and structural evolution of UiO-based photocatalysts, as well as the dynamics of charge carriers.
(3) Developing highly selective catalysts for the production of specific single products has always been a key goal in photocatalytic CO2RR. However, for most UiO-MOF-based catalysts, CO is usually the main product, often accompanied by by-products such as CH4. Therefore, there is a strong demand to achieve selective control over the generation of specific single products through sophisticated catalyst design, particularly by fine tuning of the local structure of catalytic active sites and the band structure of the photocatalyst.
(4) Most research in this area is conducted at the lab scale, typically using an Xe lamp as the excitation source, and simple target substances allow UiO-based photocatalysts to perform their catalytic function under relatively mild conditions. However, it is important to note that the photocatalytic performance of these catalysts under actual sunlight, in the presence of other ions, varying pH values, and complex environments, may differ significantly from lab results. Additionally, most UiO-based photocatalysts are used in powder form with catalytic systems, which poses challenges for recycling efficiency and stability in large-scale applications.
Furthermore, for practical applications, UiO-MOFs generally face challenges such as complex synthesis process, the use of large amounts of toxic organic solvents during synthesis, relatively high production cost, and the need to improve stability, recyclability, as well as acid and alkali resistance. To address these issues, it is necessary to develop synthesis methods that are environmentally friendly, energy-efficient, simple, capable of producing high crystallinity materials, and suitable for large-scale production.
Authors contribution
Shi L: Data analysis and interpretation, article conception and design, writing-original draft.
Xin T: Investigation, formal analysis.
Peng C, Wu Z: Formal analysis, investigation.
Liu S: Article conception, supervision.
Yu K, Lou LL: Article conception and design, supervision, writing-review & editing.
Conflicts of interest
There are no conflicts to declare.
Ethical approval
Not applicable.
Consent to participate
Not applicable.
Consent for publication
Not applicable.
Availability of data and material
Not applicable.
Funding
This work was supported by the National Key Research and Development Program of China (Grant No. 2022YFC3901401), the National Natural Science Foundation of China (Grant Nos. 22172081 and U23A20125), the Science and Technology Project of Tianjin (Grant Nos. 21ZXCCSN00010 and 23JCYBJC01820), NCC Fund (Grant NCC2020PY02), and the Fundamental Research Funds for the Central Universities.
Copyright
© The Author(s) 2025.
References
-
1. Olivier JGJ, Peters JAHW. Trends in global CO2 and total greenhouse gas emission: 2019 report. The Hague: PBL Netherlands Environmental Assessment Agency. 2019. Available from: https://www.pbl.nl/
-
2. Guan D, Meng J, Reiner DM, Zhang N, Shan Y, Mi Z, et al. Structural decline in China’s CO2 emissions through transitions in industry and energy systems. Nature Geosci. 2018;11(8):551-555.
[DOI] -
3. Li R, Zhang W, Zhou K. Metal-organic-framework-based catalysts for photoreduction of CO2. Adv Mater. 2018;30(35):1705512.
[DOI] -
4. Li D, Kassymova M, Cai X, Zhang SQ, Jiang HL. Photocatalytic CO2 reduction over metal-organic framework-based materials. Coord Chem Rev. 2020;412:213262.
[DOI] -
5. Dao XY, Sun WY. Single- and mixed-metal-organic framework photocatalysts for carbon dioxide reduction. Inorg Chem Front. 2021;8(13):3178-3204.
[DOI] -
6. Guo F, Zhao YF, Li RX, Xu H, Sun WY. Photo/electrocatalytic reduction of CO2C to C2+ products on MOF-based catalysts. ChemNanoMat. 2023;9(12):e202300313.
[DOI] -
7. Li P, Liu L, An W, Wang H, Cui W. Efficient photothermal catalytic CO2 reduction to CH3CH2OH over Cu2O/g-C3N4 assisted by ionic liquids. Appl Surf Sci. 2021;565:150448.
[DOI] -
8. Yang Y, Zhang HY, Wang Y, Shao LH, Fang L, Dong H, et al. Integrating enrichment, reduction, and oxidation sites in one system for artificial photosynthetic diluted CO2 reduction. Adv Mater. 2023;35(40):2304170.
[DOI] -
9. Wu QJ, Liang J, Huang YB, Cao R. Thermo-, electro-, and photocatalytic CO2 conversion to value-added products over porous metal/covalent organic frameworks. Acc Chem Res. 2022;55(20):2978-2997.
[DOI] -
10. Nguyen HL, Alzamly A. Covalent organic frameworks as emerging platforms for CO2 photoreduction. ACS Catal. 2021;11(15):9809-9824.
[DOI] -
11. Liu Y, Zhang M, Lu M, Lan Y. Covalent organic frameworks for photocatalytic CO2 reduction. Prog Chem. 2023;35(3):349-359.
[DOI] -
12. Guo RT, Wang J, Bi ZX, Chen X, Hu X, Pan WG. Recent advances and perspectives of core-shell nanostructured materials for photocatalytic CO2 reduction. Small. 2023;19(9):2206314.
[DOI] -
13. Wang J, Guo RT, Bi ZX, Chen X, Hu X, Pan WG. A review on TiO2-x-based materials for photocatalytic CO2 reduction. Nanoscale. 2022;14(32):11512-11528.
[DOI] -
14. Patial S, Kumar R, Raizada P, Singh P, Le QV, Lichtfouse E, et al. Boosting light-driven CO2 reduction into solar fuels: Mainstream avenues for engineering ZnO-based photocatalysts. Environ Res. 2021;197:111134.
[DOI] -
15. Su Q, Zuo C, Liu M, Tai X. A review on Cu2O-based composites in photocatalysis: Synthesis, modification and applications. Molecules. 2023;28(14):5576.
[DOI] -
16. Wu J, Liu J, Xia W, Ren YY, Wang F. Advances on photocatalytic CO2 reduction based on CdS and CdSe nano-semiconductors. Acta Phys-Chim Sin. 2021;37(5):2008043.
[DOI] -
17. Hussain MZ, Yang Z, Huang Z, Jia Q, Zhu Y, Xia Y. Recent advances in metal-organic frameworks derived nanocomposites for photocatalytic applications in energy and environment. Adv Sci. 2021;8(14):2100625.
[DOI] -
18. Sun K, Qian Y, Jiang HL. Metal-organic frameworks for photocatalytic water splitting and CO2 reduction. Angew Chem Int Ed. 2023;62(15):e202217565.
[DOI] -
19. Liu S, Zhang C, Sun Y, Chen Q, He L, Zhang K, et al. Design of metal-organic framework-based photocatalysts for hydrogen generation. Coord Chem Rev. 2020;413:213266.
[DOI] -
20. Cavka JH, Jakobsen S, Olsbye U, Guillou N, Lamberti C, Bordiga S, et al. A new zirconium inorganic building brick forming metal organic frameworks with exceptional stability. J Am Chem Soc. 2008;130(42):13850-13851.
[DOI] -
21. Zhang L, Zhang J. Metal-organic frameworks for CO2 photoreduction. Front Energy. 2019;13(2):221-250.
[DOI] -
22. Ahmadijokani F, Molavi H, Rezakazemi M, Tajahmadi S, Bahi A, Ko F, et al. UiO-66 metal-organic frameworks in water treatment: A critical review. Prog Mater Sci. 2022;125:100904.
[DOI] -
23. Liu H, Cheng M, Liu Y, Zhang G, Li L, Du L, et al. Modified UiO-66 as photocatalysts for boosting the carbon-neutral energy cycle and solving environmental remediation issues. Coord Chem Rev. 2022;458:214428.
[DOI] -
24. Calik FD, Erdogan B, Yilmaz E, Saygi G, Cakicioglu-Ozkan F. Photocatalytic degradation of aquatic organic pollutants with Zn- and Zr-based metal-organic frameworks: ZIF-8 and UiO-66. Turk J Chem. 2022;46(5):1358-1375.
[DOI] -
25. Vahabi AH, Norouzi F, Sheibani E, Rahimi-Nasrabadi M. Functionalized Zr-UiO-67 metal-organic frameworks: Structural landscape and application. Coord Chem Rev. 2021;445:214050.
[DOI] -
26. Zhou J, Gu S, Xiang Y, Xiong Y, Liu G. UiO-67: A versatile metal-organic framework for diverse applications. Coord Chem Rev. 2025;526:216354.
[DOI] -
27. Wang FX, Wang CC, Wang P, Xing BC. Syntheses and applications of UiO series of MOFs. Chinese J Inorg Chem. 2017;33(5):713-737.
[DOI] -
28. Ye X, Liu D. Metal-organic framework UiO-68 and its derivatives with sufficiently good properties and performance show promising prospects in potential industrial applications. Cryst Growth Des. 2021;21(8):4780-4804.
[DOI] -
29. Hu Z, Peng Y, Kang Z, Qian Y, Zhao D. A modulated hydrothermal (MHT) approach for the facile synthesis of UiO-66-type MOFs. Inorg Chem. 2015;54(10):4862-4868.
[DOI] -
30. Ge J, Liu L, Shen Y. Facile synthesis of amine-functionalized UiO-66 by microwave method and application for methylene blue adsorption. J Porous Mater. 2017;24(3):647-655.
[DOI] -
31. Vakili R, Xu S, Al-Janabi N, Gorgojo P, Holmes SM, Fan X. Microwave-assisted synthesis of zirconium-based metal organic frameworks (MOFs): Optimization and gas adsorption. Microporous Mesoporous Mater. 2018;260:45-53.
[DOI] -
32. Uzarevic K, Wang TC, Moon SY, Fidelli AM, Hupp JT, Farha OK, et al. Mechanochemical and solvent-free assembly of zirconium-based metal-organic frameworks. Chem Commun. 2016;52(10):2133-2136.
[DOI] -
33. Wei JZ, Gong FX, Sun XJ, Li Y, Zhang T, Zhao XJ, et al. Rapid and low-cost electrochemical synthesis of UiO-66-NH2 with enhanced fluorescence detection performance. Inorg Chem. 2019;58(10):6742-6747.
[DOI] -
34. Zhang T, Wei JZ, Sun XJ, Zhao XJ, Tang HL, Yan H, et al. Continuous and rapid synthesis of UiO-67 by electrochemical methods for the electrochemical detection of hydroquinone. Inorg Chem. 2020;59(13):8827-8835.
[DOI] -
35. Sheng B, Li C, Liu Y, Wang A, Wang Y, Zhang J, et al. Microfluidic synthesis of UiO-66 metal-organic frameworks modified with different functional groups. Chem J Chinese Universities. 2019;40(7):1365-1373.
[DOI] -
36. Yu H, Guo S, Jia M, Jia J, Chang Y, Wang J. Remarkable CO2 photocatalytic reduction enabled by UiO-66-NH2 anchored on flower-like ZnIn2S4. Arab J Chem. 2024;17(10):105975.
[DOI] -
37. Hong LF, Guo RT, Zhang ZW, Yuan Y, Ji XY, Lin ZD, et al. Fabrication of porous octahedron-flowerlike microsphere NH2-UiO-66/CdIn2S4 heterojunction photocatalyst for enhanced photocatalytic CO2 reduction. J CO Util. 2021;51:101650.
[DOI] -
38. Li D, Zhu B, Sun Z, Liu Q, Wang L, Tang H. Construction of UiO-66/Bi4O5Br2 type-II heterojunction to boost charge transfer for promoting photocatalytic CO2 reduction performance. Front Chem. 2021;9:804204.
[DOI] -
39. Tu J, Zeng X, Xu F, Wu X, Tian Y, Hou X, et al. Microwave-induced fast incorporation of titanium into UiO-66 metal-organic frameworks for enhanced photocatalytic properties. Chem Commun. 2017;53(23):3361-3364.
[DOI] -
40. Xiong W, Ouyang W, Li M, Song H, Zhao C, Hu M, et al. Highly stable lead-free perovskite Cs2AgBiBr6/UiO-66 Z-scheme heterostructures with enhanced photocatalytic activity for CO2 photoreduction and organic dyes degradation. Appl Surf Sci. 2024;644:158807.
[DOI] -
41. Zhou L, Liu F, Wang J, Chen R, Chen Y. Effects of ligand functionalization on the band gaps and luminescent properties of a Zr12 oxo-cluster based metal-organic framework. CrystEngComm. 2021;23(16):2961-2967.
[DOI] -
42. Mu F, Cai Q, Hu H, Wang J, Wang Y, Zhou S, et al. Construction of 3D hierarchical microarchitectures of Z-scheme UiO-66-(COOH)2/ZnIn2S4 hybrid decorated with non-noble MoS2 cocatalyst: A highly efficient photocatalyst for hydrogen evolution and Cr(VI) reduction. Chem Eng J. 2020;384:123352.
[DOI] -
43. Silva CG, Luz I, Xamena FXLI, Corma A, García H. Water stable Zr-benzenedicarboxylate metal-organic frameworks as photocatalysts for hydrogen generation. Chem Eur J. 2010;16(36):11133-11138.
[DOI] -
44. Sun D, Fu Y, Liu W, Ye L, Wang D, Yang L, et al. Studies on photocatalytic CO2 reduction over NH2-Uio-66(Zr) and its derivatives: towards a better understanding of photocatalysis on metal-organic frameworks. Chem Eur J. 2013;19(42):14279-14285.
[DOI] -
45. Chen TF, Han SY, Wang ZP, Gao H, Wang LY, Deng YH, et al. Modified UiO-66 frameworks with methylthio, thiol and sulfonic acid function groups: The structure and visible-light-driven photocatalytic property study. Appl Catal B Environ. 2019;259:118047.
[DOI] -
46. Hendrickx K, Vanpoucke DEP, Leus K, Lejaeghere K, Van Yperen-De, Van Speybroeck, et al. Understanding intrinsic light absorption properties of UiO-66 frameworks: A combined theoretical and experimental study. Inorg Chem. 2015;54(22):10701-10710.
[DOI] -
47. Wang K, Yan B, Zhou B, Zhang Y, Lin GL, Zhang TS, et al. Acceleration of photoinduced electron transfer by modulating electronegativity of substituents in stable Zr-metal-organic frameworks to boost photocatalytic CO2 reduction. ACS Appl Mater Interfaces. 2024;16(26):33601-33610.
[DOI] -
48. Wei YP, Liu Y, Guo F, Dao XY, Sun WY. Different functional group modified zirconium frameworks for the photocatalytic reduction of carbon dioxide. Dalton Trans. 2019;48(23):8221-8226.
[DOI] -
49. Zeama M, Morsy M, Abdel-Azeim S, Abdelnaby M, Alloush A, Yamani Z. Photophysical and photocatalytic properties of structurally modified UiO-66. Inorganica Chim Acta. 2020;501:119287.
[DOI] -
50. Sun D, Liu W, Qiu M, Zhang Y, Li Z. Introduction of a mediator for enhancing photocatalytic performance via post-synthetic metal exchange in metal-organic frameworks (MOFs). Chem Commun. 2015;51(11):2056-2059.
[DOI] -
51. Lee Y, Kim S, Kang JK, Cohen SM. Photocatalytic CO2 reduction by a mixed metal (Zr/Ti), mixed ligand metal-organic framework under visible light irradiation. Chem Commun. 2015;51(26):5735-5738.
[DOI] -
52. Cabrero-Antonino M, Melillo A, Montero-Lanzuela E, Álvaro M, Ferrer B, Vayá I, et al. Solar-driven gas phase photocatalytic CO2 methanation by multimetallic UiO-66 solids decorated with RuOx nanoparticles. Chem Eng J. 2023;468:143553.
[DOI] -
53. Asiri M, Altalbawy FMA, Sead FF, Makasana J, Rekha MM, Pathak PK, et al. Hetero bimetallic Zr-O-Cu in UiO-67 MOF for selective and efficient CO2 photoreduction to ethylene. J Mol Struct. 2025;1328:141306.
[DOI] -
54. Du XD, Yi XH, Wang P, Zheng W, Deng J, Wang CC. Robust photocatalytic reduction of Cr(VI) on UiO-66-NH2(Zr/Hf) metal-organic framework membrane under sunlight irradiation. Chem Eng J. 2019;356:393-399.
[DOI] -
55. Ma X, Wang L, Zhang Q, Jiang HL. Switching on the photocatalysis of metal-organic frameworks by engineering structural defects. Angew Chem Int Edit. 2019;58(35):12175-12179.
[DOI] -
56. Koutsianos A, Kazimierska E, Barron AR, Taddei M, Andreoli E. A new approach to enhancing the CO2 capture performance of defective UiO-66 via post-synthetic defect exchange. Dalton Trans. 2019;48(10):3349-3359.
[DOI] -
57. Fu Y, Wu J, Du R, Guo K, Ma R, Zhang F, et al. Temperature modulation of defects in NH2-UiO-66(Zr) for photocatalytic CO2 reduction. RSC Adv. 2019;9(65):37733-37738.
[DOI] -
58. Jagadeeswararao M, Swarnkar A, Markad GB, Nag A. Defect-mediated electron-hole separation in colloidal Ag2S-AgInS2 hetero dimer nanocrystals tailoring luminescence and solar cell properties. J Phys Chem C. 2016;120(34):19461-19469.
[DOI] -
59. Zhou W, Fu H. Defect-mediated electron-hole separation in semiconductor photocatalysis. Inorg Chem Front. 2018;5(6):1240-1254.
[DOI] -
60. Bueken B, Van Velthoven, Krajnc A, Smolders S, Taulelle F, Mellot-Draznieks C, et al. Tackling the defect conundrum in UiO-66: A mixed-linker approach to engineering missing linker defects. Chem Mater. 2017;29(24):10478-10486.
[DOI] -
61. Feng Y, Chen Q, Cao M, Ling N, Yao J. Defect-tailoring and titanium substitution in metal-organic framework UiO-66-NH2 for the photocatalytic degradation of Cr(VI) to Cr(III). ACS Appl Nano Mater. 2019;2(9):5973-5980.
[DOI] -
62. Ye G, Zhang D, Li X, Leng K, Zhang W, Ma J, et al. Boosting catalytic performance of metal-organic framework by increasing the defects via a facile and green approach. ACS Appl Mater Interfaces. 2017;9(40):34937-34943.
[DOI] -
63. Wang SQ, Wang X, Zhang XY, Chen XM, Ma J, Sun WY. Effect of the defect modulator and ligand length of metal-organic frameworks on carbon dioxide photoreduction. ACS Appl Mater Interfaces. 2021;13(51):61578-61586.
[DOI] -
64. Wang SQ, Gu X, Wang X, Zhang XY, Dao XY, Cheng XM, et al. Defect-engineering of Zr(IV)-based metal-organic frameworks for regulating CO2 photoreduction. Chem Eng J. 2022;429:132157.
[DOI] -
65. Shearer GC, Vitillo JG, Bordiga S, Svelle S, Olsbye U, Lillerud KP. Functionalizing the defects: Postsynthetic ligand exchange in the metal organic framework UiO-66. Chem Mater. 2016;28(20):7190-7193.
[DOI] -
66. Wang SQ, Wang X, Cheng XM, Ma J, Sun WY. Tailoring defect-type and ligand-vacancies in Zr(Ⅳ) frameworks for CO2 photoreduction. J Mater Chem A. 2022;10(31):16396-16402.
[DOI] -
67. Zhao X, Xu M, Song X, Liu X, Zhou W, Wang H, et al. Tailored linker defects in UiO-67 with high ligand-to-metal charge transfer toward efficient photoreduction of CO2. Inorg Chem. 2022;61(3):1765-1777.
[DOI] -
68. Dong YL, Liu HR, Wang SM, Guan GW, Yang QY. Immobilizing isatin-Schiff base complexes in NH2-UiO-66 for highly photocatalytic CO2 reduction. ACS Catal. 2023;13(4):2547-2554.
[DOI] -
69. Wang G, He CT, Huang R, Mao J, Wang D, Li Y. Photoinduction of Cu single atoms decorated on UiO-66-NH2 for enhanced photocatalytic reduction of CO2 to liquid fuels. J Am Chem Soc. 2020;142(45):19339-19345.
[DOI] -
70. Chu M, Cui K, Zhou Y, Yuan X, Qin W, Li Y, et al. Water-activation-facilitated photocatalytic CO2 reduction by a heterogeneous synergetic single-atomic Zn sites and Pd nanocrystals. J Alloys Compd. 2024;976:173112.
[DOI] -
71. Al-dolaimy F, Kzar MH, Hussein SA, Bahir H, Hamoody AHM, Dawood AH, et al. Incorporating of cobalt into UiO-67 metal-organic framework for catalysis CO2 transformations: An efficient bi-functional approach for CO2 insertion and photocatalytic reduction. J Inorg Organomet Polym. 2024;34(2):864-873.
[DOI] -
72. Pan W, Wei Z, Su Y, Lian Y, Zheng Z, Yuan H, et al. Hydroxylated metal-organic-layer nanocages anchoring single atomic cobalt sites for robust photocatalytic CO2 reduction. Nano Res. 2024;17:2410-2419.
[DOI] -
73. Wang C, Xie Z, deKrafft KE, Lin W. Doping metal-organic frameworks for water oxidation, carbon dioxide reduction, and organic photocatalysis. J Am Chem Soc. 2011;133(34):13445-13454.
[DOI] -
74. Liao WM, Zhang JH, Wang Z, Yin SY, Pan M, Wang HP, et al. Post-synthetic exchange (PSE) of UiO-67 frameworks with Ru/Rh half-sandwich units for visible-light-driven H2 evolution and CO2 reduction. J Mater Chem A. 2018;6(24):11337-11345.
[DOI] -
75. Gao X, Guo B, Guo C, Meng Q, Liang J, Liu J. Zirconium-based metal-organic framework for efficient photocatalytic reduction of CO2 to CO: The influence of doped metal ions. ACS Appl Mater Interfaces. 2020;12(21):24059-24065.
[DOI] -
76. Wang J, Sun K, Wang D, Niu X, Lin Z, Wang S, et al. Precise regulation of the coordination environment of single Co(II) sites in a metal-organic framework for boosting CO2 photoreduction. ACS Catal. 2023;13(13):8760-8769.
[DOI] -
77. Wang X, Su Y, Yang G, Chai G, Xu Z, Nasir MS, et al. Synthesis of Au/UiO-66-NH2/Graphene composites as efficient visible-light photocatalysts to convert CO2. Int J Hydrogen Energy. 2021;46(21):11621-11635.
[DOI] -
78. Xiao JD, Shang Q, Xiong Y, Zhang Q, Luo Y, Yu SH, et al. Boosting photocatalytic hydrogen production of a metal-organic framework decorated with platinum nanoparticles: The platinum location matters. Angew Chem Int Edit. 2016;55(32):9389-9393.
[DOI] -
79. Guo F, Wei YP, Wang SQ, Zhang XY, Wang FM, Sun WY. Pt nanoparticles embedded in flowerlike NH2-UiO-68 for enhanced photocatalytic carbon dioxide reduction. J Mater Chem A. 2019;7(46):26490-26495.
[DOI] -
80. Chen J, Su Y, Wang Y, Wei Z, Zheng Z, Yuan H, et al. Ultrahigh loading of confined plasmonic nanoparticles within thiol-functionalized metal-organic frameworks for efficient photocatalytic CO2 reduction to CO and hydrocarbons. Nano Res. 2025;18(2):94907181.
[DOI] -
81. Hu S, Deng Z, Xing M, Wu S, Zhang J. Highly dispersed cobalt centers on UiO-66-NH2 for photocatalytic CO2 reduction. Catal Lett. 2023;153(5):1475-1482.
[DOI] -
82. Elsafi A, Theihmed Z, Al-Yafei A, Alkhateeb A, Abotaleb A, Anwar M, et al. Plasmon-enhanced CO2 reduction to liquid fuel via modified UiO-66 photocatalysts. Catalysts. 2025;15(1):70.
[DOI] -
83. Wang HN, Zou YH, Fu YM, Meng X, Xue L, Sun HX, et al. Integration of zirconium-based metal-organic framework with CdS for enhanced photocatalytic conversion of CO2 to CO. Nanoscale. 2021;13(40):16977-16985.
[DOI] -
84. Ding L, Bai F, Borjigin B, Li Y, Li H, Wang X. Embedding Cs2AgBiBr6 QDs into Ce-UiO-66-H to in situ construct a novel bifunctional material for capturing and photocatalytic reduction of CO2. Chem Eng J. 2022;446:137102.
[DOI] -
85. Liu J, Qin W, Wang Y, Xu Q, Xie Y, Chen Y, et al. NH2-UiO-66 modification BiOBr enhancement photoreduction CO2 to CO. Sep Purif Technol. 2024;344:127289.
[DOI] -
86. Li N, Ma YL, Zhang HJ, Zhou DY, Yao BL, Wu JF, et al. Disentangling the efficient photocatalytic reduction of CO2 by a stable UiO-66-NH2/Cs2AgBiBr6 catalyst. Mater Today Chem. 2024;41:102306.
[DOI] -
87. Kong N, Du H, Li Z, Lu T, Xia S, Tang Z, et al. Nano heterojunction of double MOFs for improved CO2 photocatalytic reduction performance. Colloids Surf A Physicochem Eng Asp. 2023;663:131005.
[DOI] -
88. Yan ZH, Ma B, Li SR, Liu J, Chen R, Du MH, et al. Encapsulating a Ni(II) molecular catalyst in photoactive metal-organic framework for highly efficient photoreduction of CO2. Sci Bull. 2019;64(14):976-985.
[DOI] -
89. Tan L, Li Y, Lv Q, Gan Y, Fang Y, Tang Y, et al. Development of soluble UiO-66 to improve photocatalytic CO2 reduction. Catal Today. 2023;410:282-288.
[DOI] -
90. Wang X, Yang G, Chai G, Nasir MS, Wang S, Zheng X, et al. Fabrication of heterostructured UIO-66-NH2/CNTs with enhanced activity and selectivity over photocatalytic CO2 reduction. Int J Hydrogen Energy. 2020;45(55):30634-30646.
[DOI] -
91. Wan S, Ou M, Zhong Q, Wang X. Perovskite-type CsPbBr3 quantum dots/UiO-66(NH2) nanojunction as efficient visible-light-driven photocatalyst for CO2 reduction. Chem Eng J. 2019;358:1287-1295.
[DOI] -
92. Liu X, Zhu C, Li M, Xing H, Zhu S, Liu X, et al. Confinement synthesis of atomic copper-anchored polymeric carbon nitride in crystalline UiO-66-NH2 for high-performance CO2-to-CH3OH photocatalysis. Angew Chem Int Edit. 2024;63(45):e202412408.
[DOI] -
93. Ma X, Zhang Y, Zhou A, Jia Y, Xie Z, Ding L, et al. Modulation of interface structure on titanium-based metal-organic frameworks heterojunctions for boosting photocatalytic carbon dioxide reduction. J Colloid Interface Sci. 2025;685:696-705.
[DOI] -
94. Yu F, Chen L, Shen X, Li X, Duan C. NH2-UiO-66/g-C3N4/CdTe composites for photocatalytic CO2 reduction under visible light. APL Mater. 2019;7(10):101101.
[DOI] -
95. Wang W, Song S, Wang P, He M, Fang Z, Yuan X, et al. Chemical bonding of g-C3N4/UiO-66(Zr/Ce) from Zr and Ce single atoms for efficient photocatalytic reduction of CO2 under visible light. ACS Catal. 2023;13(7):4597-4610.
[DOI] -
96. Yuan N, Mei Y, Liu Y, Xie Y, Lin B, Zhou Y. Fabrication of UiO-66-NH2/Ce(HCOO)3 heterojunction with enhanced photocatalytic reduction of CO2 to CH4. J CO Util. 2022;64:102151.
[DOI] -
97. Wang SQ, Zhang XY, Dao XY, Cheng XM, Sun WY. Cu2O@Cu@UiO-66-NH2 ternary nanocubes for photocatalytic CO2 reduction. ACS Appl Nano Mater. 2020;3(10):10437-10445.
[DOI] -
98. Liu N, Hu B, Tang K, Xia T, Li F, Quan G, et al. Assembling UiO-66 into layered HTiNbO5 nanosheets for efficient photocatalytic CO2 reduction. Surf Interfaces. 2023;41:103134.
[DOI] -
99. Chen L, Yu F, Shen X, Duan C. N-CND modified NH2-UiO-66 for photocatalytic CO2 conversion under visible light by a photo-induced electron transfer process. Chem Commun. 2019;55(33):4845-4848.
[DOI] -
100. Zhang H, Ye JH, Dai YH, Yang HY, Zhang JS, Xie Y, et al. Ce4+/Ce3+ redox effect-promoted UiO-66(Ce)/BiOBr heterojunction for enhancement photoreduction CO2 under visible light. Chem Eng J. 2024;502:158240.
[DOI] -
101. Zhao X, Sun L, Jin X, Xu M, Yin S, Li J, et al. Cu media constructed Z-scheme heterojunction of UiO-66-NH2/Cu2O/Cu for enhanced photocatalytic induction of CO2. Appl Surf Sci. 2021;545:148967.
[DOI] -
102. Yang X, Wang T, Ma H, Shi W, Xia Z, Yang Q, et al. Matched micro-geometrical configuration leading to hetero-interfacial intimate contact of MoS2@UiO-66-NH2 Z-scheme heterojunction for efficient photocatalytic CO2 reduction. J Mater Sci Technol. 2024;182:210-219.
[DOI] -
103. Wang J, Dai Z, Wang L, Zhang D, Wang Y, Li J, et al. A Z-scheme heterojunction of porphyrin-based core-shell Zr-MOF@Pro-COF-Br hybrid materials for efficient visible-light-driven CO2 reduction. J Mater Chem A. 2023;11(4):2023-2030.
[DOI] -
104. Dehghani Z, Jangi SRH. Novel nanophotocatalytic double Z-scheme CdS/Ag-AgBr/Au-loaded amino-defective-UiO-66(Ce) for rapid high throughput reusable photocatalytic methanol synthesis from CO2. Fuel. 2025;385:134139.
[DOI] -
105. Liu H, Yang Y, Guo C, Zhou Y. Fabrication of a direct Z-scheme heterojunction of UiO-66-NH2 and tubular g-C3N4 for the stable photocatalytic reduction of CO2 to CO and CH4. Catal Sci Technol. 2024;14(20):5938-5948.
[DOI] -
106. Liu H, Xie Y, Li Y, Zhou Y. Construction of Z-scheme heterojunction between Zn-doped UiO-66-NH2 and CeO2 towards highly selective photoreduction of CO2 to CH4. J Mater Chem C. 2024;12(24):8909-8923.
[DOI] -
107. Zhang P, Wang T, Ma H, Ma R, Xia Z, Yang Q, et al. Heterointerface connection with multiple hydrogen-bonding in Z-scheme heterojunction SiW9Co3@UiO-67-NH2 deciding high stability and photocatalytic CO2 reduction performance. Inorg Chem. 2023;62(49):20401-20411.
[DOI] -
108. Quach TA, Gopalakrishnan VN, Becerra J, Nguyen DT, Ahad JME, Mohan S, et al. Z-scheme heterojunction of chemically integrated COF-366-Co/UiO-66-NH2 MOFs nanocomposites for selective production of CO via CO2 solar-drive photoreduction. Catal Today. 2023;421:114218.
[DOI] -
109. Guo H, Zhang T, Ma W, Cheng S, Ding J, Zhong Q, et al. Construction of sandwich-like Ag/UiO-66@g-C3N4 Z-scheme ternary heterojunction for photocatalytic CO2 conversion to CH3OH and CO. Fuel. 2023;344:127911.
[DOI] -
110. Zhang M, Wang X, Qi X, Guo H, Liu L, Zhao Q, et al. Effect of Ag cocatalyst on highly selective photocatalytic CO2 reduction to HCOOH over CuO/Ag/UiO-66 Z-scheme heterojunction. J Catal. 2022;413:31-47.
[DOI] -
111. Mei Y, Yuan N, Xie Y, Li Y, Lin B, Zhou Y. Facilely fabrication of the direct Z-scheme heterojunction of NH2-UiO-66 and CeCO3OH for photocatalytic reduction of CO2 to CO and CH4. Appl Surf Sci. 2022;597:153725.
[DOI] -
112. Meng J, Chen Q, Lu J, Liu H. Z-Scheme photocatalytic CO2 reduction on a heterostructure of oxygen-defective ZnO/reduced graphene oxide/UiO-66-NH2 under visible light. ACS Appl Mater Interfaces. 2019;11(1):550-562.
[DOI] -
113. Zhang L, Zhang J, Yu H, Yu J. Emerging S-scheme photocatalyst. Adv Mater. 2022;34(11):2107668.
[DOI] -
114. Li S, Li H, Wang Y, Liang Q, Zhou M, Guo D, et al. Mixed-valence bimetallic Ce/Zr-NH2-UiO-66 modified with CdIn2S4 to form S-scheme heterojunction for boosting photocatalytic CO2 reduction. Sep Purif Technol. 2024;333:125994.
[DOI] -
115. Zhao X, Xu M, Song X, Zhou W, Liu X, Yan Y, et al. Charge separation and transfer activated by covalent bond in UiO-66-NH2/RGO heterostructure for CO2 photoreduction. Chem Eng J. 2022;437:135210.
[DOI] -
116. Fang Z, Yue X, Xiang Q. Atomically contacted Cs3Bi2Br9 QDs@UiO-66 composite for photocatalytic CO2 reduction. Small. 2024;20(34):2401914.
[DOI] -
117. Sun J, Guan Y, Yang G, Qiu S, Shao H, Wang Y, et al. S-scheme photocatalyst NH2-UiO-66/CuZnS with enhanced photothermal-assisted CO2 reduction performances. ACS Sustainable Chem Eng. 2023;11(40):14827-14840.
[DOI] -
118. Chen L, Tang Q, Wu S, Zhang L, Feng L, Wang Y, et al. Covalent coupling promoting charge transport of CdSeTe/UiO-66 for boosting photocatalytic CO2reduction. Chin Chem Lett. 2023;34(6):107903.
[DOI] -
119. Niu Q, Dong S, Tian J, Huang G, Bi J, Wu L. Rational design of novel COF/MOF S-scheme heterojunction photocatalyst for boosting CO2 reduction at gas-solid interface. ACS Appl Mater Interfaces. 2022;14(21):24299-24308.
[DOI] -
120. Xiao S, Guan Y, Shang H, Li H, Tian Z, Liu S, et al. An S-scheme NH2-UiO-66/SiC photocatalyst via microwave synthesis with improved CO2 reduction activity. J CO Util. 2022;55:101806.
[DOI] -
121. Wang X, Wang Y, Chai G, Yang G, Wang C, Yan W. Poly(triphenylamine)-decorated UiO-66-NH2 mesoporous architectures with enhanced photocatalytic activity for CO2 reduction and H2 evolution. J Util. 2021;51:101654.
[DOI] -
122. Wang X, Zhao X, Zhang D, Li G, Li H. Microwave irradiation induced UiO-66-NH2 anchored on graphene with high activity for photocatalytic reduction of CO2. Appl Catal B Environ. 2018;228:47-53.
[DOI] -
123. Saadh MJ, Mustafa MA, Altalbawy FMA, Ballal S, Prasad GVS, Al-saray MJ, et al. Integration TiO2 nanosheets into defect engineered Zr-based MOF for highly selective and efficient photocatalytic conversion of CO2 to ethane. J Mol Struct. 2025;1325:140830.
[DOI]
Copyright
© The Author(s) 2025. This is an Open Access article licensed under a Creative Commons Attribution 4.0 International License (https://creativecommons.org/licenses/by/4.0/), which permits unrestricted use, sharing, adaptation, distribution and reproduction in any medium or format, for any purpose, even commercially, as long as you give appropriate credit to the original author(s) and the source, provide a link to the Creative Commons license, and indicate if changes were made.
Publisher’s Note
Share And Cite