Tiantian He, Institute of Additive Manufacturing, Jiangxi University of Science and Technology, Nanchang 330013, Jiangxi, China. E-mail: tiantianhe202337@163.com
Abstract
Bone defects represent a significant orthopedic challenge, with associated disorders continue to pose clinical difficulties. In the biomedical field, advancements in three-dimensional (3D) printing technology have established bone tissue engineering (BTE) scaffolds as a promising approach for effective treatment. These scaffolds not only provide structural support for cells but also serve as templates to guide bone tissue regeneration. In recent years, owing to their exceptional physicochemical properties, two-dimensional nanomaterials (2D NMs) have garnered increasing attention and have been widely explored as additives in the fabrication of BTE scaffolds. This review centers on the most recent developments in the combination of 2D NMs and 3D printing for BTE applications. It begins with a concise summary of the common synthesis and surface modification methods of 2D NMs. Then, it offers a comprehensive overview of recent advancements in their use within BTE. Finally, it discusses current challenges and future perspectives regarding the application of 2D NMs-based 3D-printed scaffolds in bone tissue regeneration.
Keywords
1. Introduction
Large bone defects caused by trauma, infection, tumor resection, and degenerative conditions have led to an increasing demand for bone-related implants for replacement or reconstructive surgeries[1,2]. In recent years, bone tissue engineering (BTE) has emerged as a promising strategy for fabricating bone graft substitutes to address these defects[3]. Ideal BTE grafts are expected to possess the following characteristics: (1) Excellent biomechanical properties, BTE grafts with appropriate mechanical strength can provide sufficient support without inducing stress shielding effect, especially in load-bearing bone tissues; (2) Outstanding biocompatibility and biodegradability, BTE grafts should provide a favorable matrix microenvironment for cell migration, adhesion, and proliferation, while minimizing the risk of immune rejection. Furthermore, the grafts should be gradually reconstructed and absorbed along with the growth of new bone; (3) Promoting of osteogenesis and angiogenesis, BTE grafts with microporous structures should be capable of incorporating osteogenic and angiogenic factors to facilitate osteogenic and angiogenic differentiation of mesenchymal stem cells (MSCs), thereby promoting vascularization and new bone generation[4-7]. As an optimal replacement strategy, artificial bone grafts have garnered increasing attention in BTE.
Three-dimensional (3D) printing, also known as rapid prototyping or additive manufacturing (AM), is a "bottom-up" fabrication technology to fabricate materials including polymers, ceramics, metals, and other composites with various shapes and sizes based on a computer-aided design[8]. The concept of 3D printing was first introduced in 1980s, when Charles Hull introduced the stereolithography technique to the world. Over the years, significant advances have been made in the evolution of 3D printing technologies, including selective laser sintering, fused deposition modeling (FDM), selective laser melting, laminated object manufacturing, inkjet 3D printing, digital light processing, electron beam melting, and direct metal laser sintering, etc.[9,10]. A key feature of 3D printing lies in its ability to precisely control overall architectures and internal structures through customized designs, which has spurred explosive growth in a variety of fields — especially in biomedical tissue engineering[11-13]. It has been constantly and comprehensively researched for BTE since the development of scaffolds based on 3D printing, particularly combined with nanoscale materials, which have extended the way for designing and manufacturing customized bone grafts with personalized shapes and internal structures.
Two-dimensional nanomaterials (2D NMs) are a novel class of nanomaterial characterized by a thickness of less than 100 nm and a significantly larger lateral dimension. The first discovered 2D NMs was single-layer graphene, exfoliated from graphite in 2004, which marked the beginning of a new era in 2D NMs[14]. In recent years, various new types of 2D NMs have been developed, including black phosphorus (BP), transition metal dichalcogenides (TMDs), transition metal carbides/nitrides (Mxenes), laponite (Lap), layered double hydroxides (LDHs), metal-organic frameworks (MOFs), antimonene nanosheets, hexagonal boron nitride nanosheets, ultrathin 2D boron (B) nanosheets, and Pd nanosheets[15-21]. Meanwhile, a variety of synthesis techniques have been established, which are generally categorized into top-down methods (e.g., mechanical cleavage, liquid-phase exfoliation) and bottom-up methods (e.g., chemical vapor deposition, hydrothermal/solvothermal synthesis, and solution-phase reactions)[22-27]. Notably, 2D NMs exhibit a range of exceptional physicochemical properties compared to their bulk counterparts, such as a high surface area-to-weight ratio, tunable surface chemical, remarkable optical and electrical performance, and excellent biocompatibility. These features have made 2D NMs highly attractive for applications in various fields, particularly in biomedical tissue engineering[28-32]. The unique wide surface area and exceptionally low cytotoxicity can support cell adhesion and proliferation in the scaffolds, appropriate mechanical properties and inherent osteogenesis characteristics facilitate directional differentiation of MSCs, which is conducive to applications in bone tissue regeneration[33-35]. Hence, it is significant to carry out the systematic review of 2D NMs combined with 3D-printed scaffolds for bone tissue regeneration, to promote the prediction of more potential applications.
In this review, we summarize recent advances in the integration of 2D NMs with 3D printing for applications in BTE. First, we outline general strategies for the synthesis and surface modification of 2D NMs. Subsequently, we present the recent progress in the use of various types of 2D NMs — such as graphene and its derivatives, BP, TMDs, Mxenes, Lap, LDHs, MOFs, and others — in combination with 3D-printed scaffolds, as illustrated in Figure 1. Finally, we discuss current challenges and future perspectives related to 2D NMs-based 3D-printed scaffolds for bone tissue regeneration, aiming to provide valuable insights into the next generation of bioactive scaffolds.
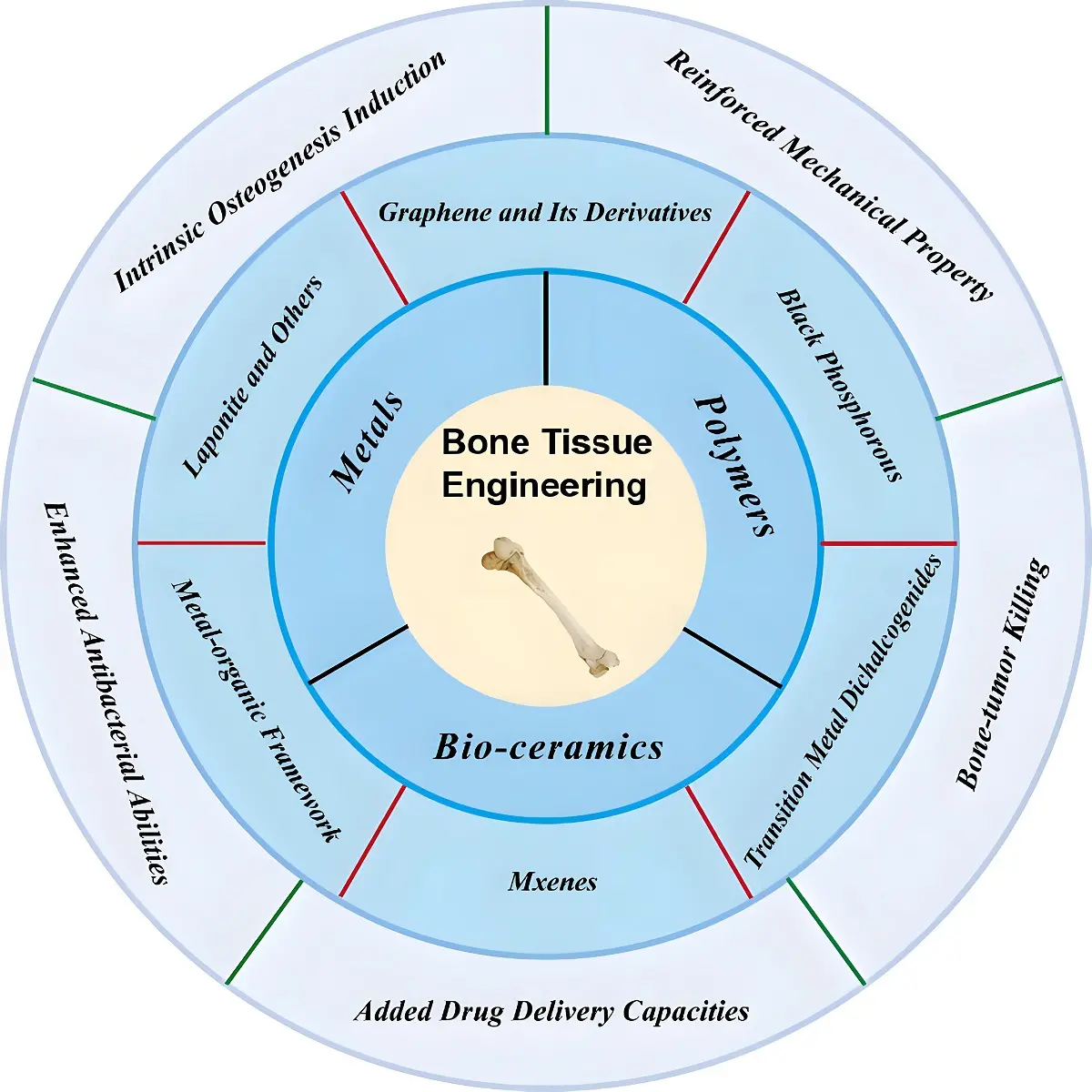
Figure 1. Various types of 2D NMs and their applications in BTE. NMs: nanomaterials; BTE: bone tissue engineering.
2. The Synthesis and Surface Modification of 2D NMs
Over the years, numerous methods have been developed for the preparation of 2D NMs, which are generally classified into two main categories: top-down and bottom-up approaches. As shown in Figure 2 and Table 1, various synthesis techniques along with their respective advantages and limitations are presented.
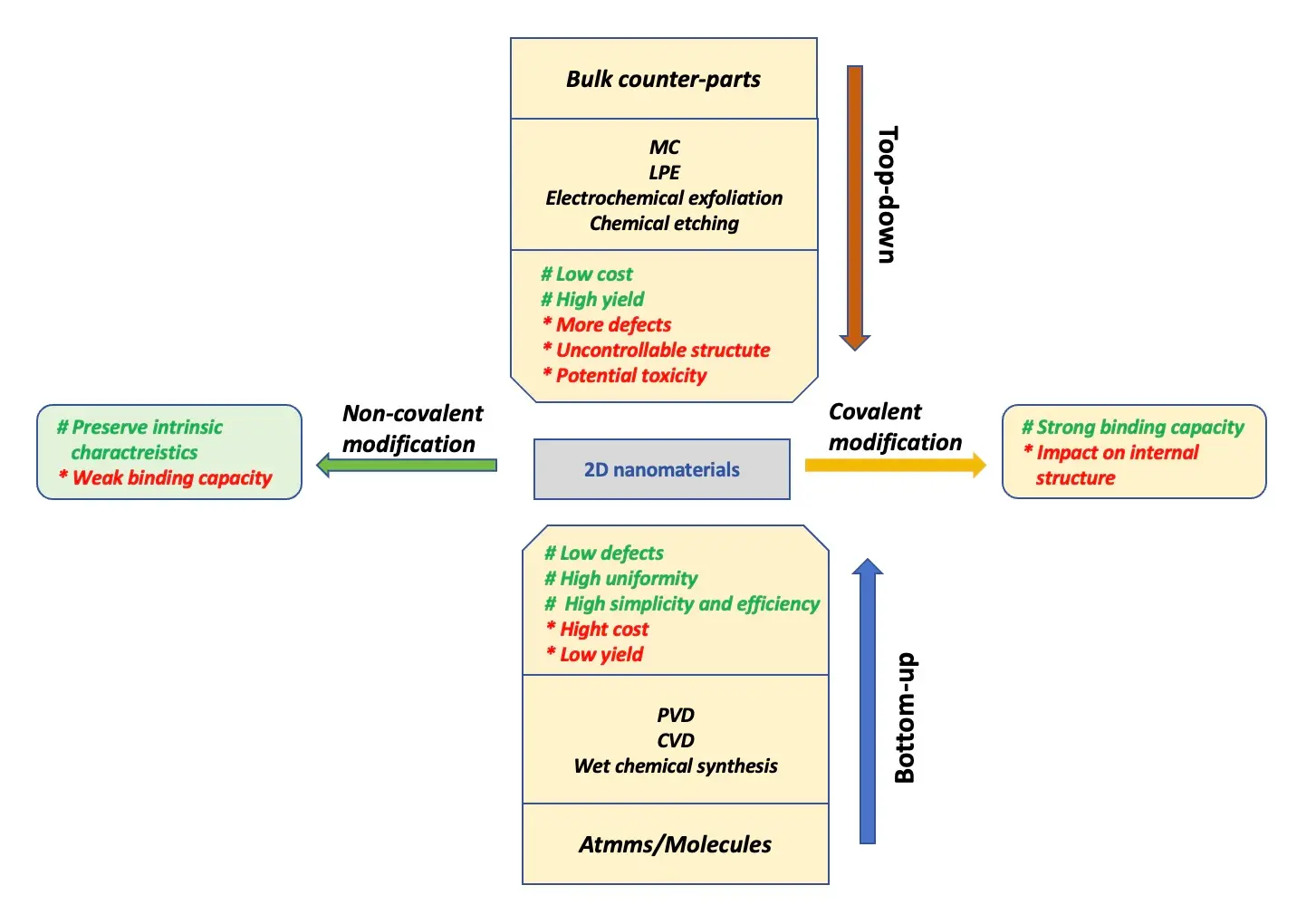
Figure 2. Various types of methods and their strengths and limitations in the synthesis of 2D NMs. NMs: nanomaterials; MC: mechanical cleavage; LPE: liquid-phase exfoliation; PVD: physical vapor deposition; CVD: chemical vapor deposition.
Method | Principle | Core parameters | Pros | Cons | Ref |
MC | Peeling atomically thin layers from bulk crystals (e.g., graphene from graphite). | Exfoliation force, Substrate adhesion. | High-quality flakes; simple setup. | Low yield; limited scalability. | [46] |
CVD | Gas-phase precursors react on substrates (e.g., Cu foil) to form 2D layers. | Temperature, Precursor ratio. | Large-area uniform films; tunable. | High temperatures; substrate-dependent. | [47] |
LPE | Sonication/shear mixing in solvents to exfoliate bulk materials (e.g., graphene). | Solvent surface energy, Ultrasonic power. | Scalable; solution-processable. | Defects/thickness variability. | [48] |
Hydrothermal | High-temperature/pressure reactions in sealed reactors to grow 2D crystals. | Reaction T, pH. | Controllable morphology; versatile. | Long reaction times; thickness control. | [49] |
Epitaxial Growth | Atomic-layer deposition on single-crystal substrates (e.g., graphene on SiC). | Substrate lattice matching, Cooling rate. | High crystallinity; interface control. | Expensive; limited substrate options. | [50] |
Self-Assembly | Molecular/ionic components organize into 2D structures (e.g., Langmuir films). | Concentration, Ionic strength. | Tunable chemistry; large-area films. | Weak interlayer bonds; defects. | [51] |
NMs: nanomaterials; MC: mechanical cleavage; CVD: chemical vapor deposition; LPE: liquid-phase exfoliation.
2.1 Top-down methods
The top-down approach is primarily applied to bulk layered materials, in which multilayered crystals are exfoliated into single- or few-layer nanosheets. The earliest technique used for preparing 2D NMs is mechanical cleavage (MC), reported in 2004 when Novoselov et al. successfully exfoliated graphite into single-layer graphene through repeat peeling[14]. In addition, ball milling is another early MC method, in which high-speed rotating grinding balls generate both normal and shear force on the surface of graphite sheets, helping to overcome the van der Waals interactions between layers[22]. This method was later extended to the preparation of other 2D nanomaterials, including hexagonal boron nitride (h-BN), MoS2, and others[36,37]. As a purely physical separation process, MC offers a direct means of obtaining 2D nanosheets with high crystallinity and purity, since no chemical reaction is involved. However, its low yields (typically around 3%-10%) and poor control over layer number have limited its potential for large-scale production[38].
Liquid-phase exfoliation (LPE) is considered one of the most effective top-down approaches for producing 2D nanosheets from multilayered materials. This method typically employs ultrasonication in a liquid medium to break apart crystals held together by weak van der Waals forces. The exfoliation process primarily relies on ultrasonic shearing forces and the interactions between the solvent and layered nanosheets[25]. For example, Hanlon et al. successfully prepared stable, few-layer BP nanosheets with controlled sizes using N-cyclohexyl-2-pyrrolidone and N-methylpyrrolidone as exfoliation solvents, which effectively isolated the reaction system from water and oxygen[18]. The LPE technique can produce homogeneous nanosheet dispersions with concentrations exceeding 1 g/L[39]. However, this approach also presents certain limitations, including demanding reaction conditions, prolonged ultrasonication times, and the potential toxicity of residual organic solvents.
In recent years, electrochemical exfoliation has been widely employed for the production of 2D nanosheets. This method involves the application of a bias voltage to drive cations or anions in the electrolyte to intercalate into the interlayer spaces of bulk materials[40]. Furthermore, microwave, ultrasound, and other means are employed to promote the yield. Eventually, the nanosheets are obtained through filtration and other purification steps. Hence, the stripping efficiency is closely related to the types of electrolyte, voltage/current, and electrode. For instance, Liu et al. utilized electrochemical exfoliation to produce high-quality MoS2 nanosheets with low oxidation levels. The lateral dimensions of the exfoliated nanosheets reached up to 50 μm, significantly larger than those produced by MC and LPE methods[23]. However, this approach also faces several limitations, including the difficulty of completely removing residual electrolyte from the final product and the challenge of precisely controlling the nanosheet structure.
In addition to the aforementioned methods, the fabrication of Mxenes commonly involves a top-down chemical etching approach, which requires the selective removal of the A layer from MAX phase precursors — hexagonal layered compounds with the general formula M n+1AXn, where M is an early transition metal, A is typically a group IIIA or IVA element (e.g., aluminum), X represents carbon and/or nitrogen, and n range from 1 to 4[41]. In 2011, Gogotsi et al. first reported the use of 50% hydrofluoric acid to synthesize MXene nanosheets. This process relied on the strong and selective interaction between fluoride ions and the A-element, leading to the disruption of M-A bonds and removal of the A-layer from MAX phase[42]. Subsequently, Ghidiu et al. introduced an improved method using a HCl/LiF solution, enabling the obtained MXene products to be readily delaminated into single layers through ultrasonication or even manual shaking, thereby simplifying the preparation process[43]. Additionally, other etchants such as HCl/NH4F, H2SO4/LiF, and NH4HF2 have also been explored for the deconstruction of multilayered MAX phases[44,45].
2.2 Bottom-up methods
The bottom-up approach involves the direct synthesis of 2D NMs from corresponding precursor. This strategy primarily includes physical vapor deposition (PVD), chemical vapor deposition (CVD), and wet chemical synthesis. PVD typically relies on the vaporization of source materials — either solid or liquid — into gaseous atoms or molecules, which are then deposited onto a substrate surface under ultra-high vacuum conditions. This technique is characterized by its relatively simple process, ability to produce large-area films with atomic-scale uniformity and high crystal phase, and environmentally friendly operation. To date, PVD has been widely applied to the synthesis of various materials. For example, high-quality ultrathin SnS films were successfully synthesized by heating SnS powder to 600 °C for 20 minutes under an argon atmosphere[27]. This technique has also been employed in the preparation of single-crystalline or few-layered nanosheets of g-C3N4, ZnTe, antimonene, and ReS2 by adjusting reaction temperature and duration[52-55].
The CVD method is a classical bottom-up approach that has been widely employed to synthesize 2D NMs with controllable size and thickness, based on chemical reactions and the deposition of gaseous precursors onto substrate surfaces under high-temperature conditions[56]. The concept of CVD was first mentioned by Powell in 1966. Subsequently, Nelson and Richardson deposited carbon films using a CO2 laser-focused beam, which opened the research of CVD[57]. Similar to PVD, CVD is essentially a gas-phase mass transfer process at the atomic level. However, it offers several advantages over PVD, including broader applicability, low cost, greater practicality, and fewer requirements regarding precursors and substrates[24]. In 2006, Prakash et al. first reported a simple thermal CVD method for synthesizing few-layer graphene on Ni substrates[58]. Wu et al. obtained nanolayered antimonene using a CVD process, in which Sb powder was used to deposit flakes on SiO2 substrates. The resulting antimonene exhibited a lateral size of approximately 40 μm and a thickness of about 0.73 nm, and it was found to be more easily oxidized compared to thicker slice (~ 23.3 nm)[59]. Besides, CVD has been broadly applied to prepare high-quality TMDs such as WS2, MoSe2, and MoS2, in the form of ultrathin films[60-62]. Nevertheless, this method has certain limitations, including the need for volatile precursors, potential environmental pollution, and toxicity concerns arising from the chemical byproducts of the reaction.
Wet-chemical methods, which rely on chemical reactions between active precursors in liquid phases under specific conditions, represent another widely used bottom-up strategy for synthesizing 2D NMs. Among these, the hydrothermal and solvothermal methods are commonly employed to fabricate 2D NMs with tunable size and thickness. In these approaches, precursors and solvents are sealed in containers and subjected to elevated temperatures and pressures for a defined period of time[63]. As a typical example, Yuki et al. used a supercritical hydrothermal method to prepare various types of MoS2 nanosheets by altering the reaction time and type of reductant. They confirmed that MoS2 nanosheets with 6-7 layers could be synthesized using formic acid as the reductant, while ultrathin MoS2 nanosheets were obtained using ascorbic acid, a less toxic alternative[26]. In another study, Yu's team successfully employed a solvothermal method combined with plant grafting technology to synthesize CoSe2/MoS2 hybrid nanomaterials, which showed potential as catalyst for electrochemical water reduction[64]. Although the high simplicity and efficiency prompt the wide application in 2D NMs of hydro/solve-thermal method, the preparation process is susceptible to being affected by reaction parameters (such as temperature, reaction time, and intensity of pressure).
2.3 Surface modification of 2D NMs
Although 2D NMs possess exceptional physicochemical properties and biocompatibility, several challenges remain to be addressed for their biomedical applications. First, The spontaneous aggregation of unmodified 2D NMs in liquid environments, such as phosphate-buffered solution, normal saline, or culture medium, is primarily attributed to their large surface area and hydrophobicity, which can result in significant cytotoxic effect[65]. Second, single 2D NMs tend to show poor stability in aqueous or oxidative environments due to the exposured lone-pair electrons or reactive surface functional groups (e.g., -F, -OH, or -O)[66,67]. Therefore, surface modification or functionalization of bare 2D NMs is essential to meet the diverse requirements of practical biomedical applications. These modifications are generally categorized into covalent and noncovalent strategies.
Covalent modification of 2D NMs primarily involves the formation of chemical bonds between 2D NMs and functional molecules or polymers, thereby enhancing their structural stability and intrinsic properties. For example, Depan et al performed covalent functionalization of physically exfoliated graphene oxide (GO) nanosheets through the bonding of the carboxyl groups (-COOH) on GO with the amine groups (-NH2) of chitosan (CS). The modified GO was then incorporated into CS-based scaffolds and cultured with osteoblasts for two weeks. The results confirmed that the low cytotoxicity and pro-osteogenic potential of the GO-CS scaffolds[68]. Polyethylene glycol (PEG), another classic covalent modifier, has been widely used to improve the dispersibility and biological stability of 2D NMs under physiological conditions. Deng et al. designed a heterobifunctional BP nano-platform (BPQD-PEG-TPP) through covalent bonding, which significantly enhanced its biostability, dispersibility, and mitochondria targeting capabilities[69]. Similarly, Xu and co-workers conjugated an aminated PEG linker with GO nanosheets via covalent bonds, creating a promising nanocarrier for paclitaxel delivery with demonstrated anti-tumor efficacy[70].
Compared to covalent modification, non-covalent strategies primarily rely on physical interactions and intermolecular interactions forces, such as van der Waals forces, hydrogen bonding, hydrophobic interactions, electrostatic attraction, and π-π stacking, which enhance the dispersibility, stability, and biocompatibility of 2D NMs without altering their structure or intrinsic properties[71]. For example, Li et al. developed a novel non-covalent π-π interaction strategy to functionalize BP nanosheets using 1-pyrene-butyric acid. The modified BP nanosheets exhibited excellent stability and electrochemical performance, while also providing a functional surface rich in carboxyl groups for anchoring biomolecules, enabling selective tumor cells killing[66]. A pH-responsive drug delivery system was constructed based on RGD-functionalized chitosan/graphene oxide, in which non-covalent interactions, primarily hydrogen bonding, between GO and doxorubicin (DOX) significantly improved the drug loading capacity (1.00 mg/mg). This system demonstrated excellent biostability under physiological conditions and an accelerated drug release rate in acidic environment[72]. It is worth noting that the binding strength of non-covalent modifications is generally weaker than that of covalent strategies. Therefore, appropriate surface modification methods should be selected based on the specific application requirements of 2D NMs.
3. The Application of 2D NMs in BTE
3.1 Graphene and its derivatives
Since it was first discovered in 2004, graphene has been extensively studied due to its remarkable intrinsic properties. Structurally, graphene consists of a single layer of carbon atoms arranged in a hexagonal, honeycomb-like lattice, where each carbon atoms is connected via covalent sp2 bonds. Its exceptionally large surface area (approximately 2,600 m2 /g) enables efficient loading of various molecules[19]. In addition, the strong C-C covalent bonds endow graphene with an increased Young's modulus and fracture strength[34]. Furthermore, π-π interactions provide a favorable platform for the functionalization of graphene with various biomolecules. The main derivatives of graphene mainly involve GO and reduced graphene oxide (rGO). GO is produced through the oxidation of graphene, while rGO is obtained by further removing its oxygen-containing functional groups from GO. Compared to graphene, GO contains more functional groups, such as hydroxyl, carboxyl, and epoxy groups, which enhance its hydrophilicity and reduce cytotoxicity through interactions with biomolecules. In contrast, rGO exhibits higher compressive strength, improved calcium absorption capacity, and greater alkaline phosphatase compared to GO[73]. Moreover, it has been demonstrated that graphene-based nanomaterials can promote the adhesion and osteogenic differentiation of mesenchymal stem cells, thereby facilitating bone formation[33,74,75]. Collectively, these properties highlight the significant biomedical potentials of graphene and its derivatives in applications such as drug delivery, bioimaging, and tissue engineering.
Combining with the above excellent characteristics, graphene and its derivatives are considered ideal candidates for applications in BTE. Up to now, graphene-based 3D printed scaffolds have rapidly advanced and remain a research focus in graphene-related bone regeneration, with numerous notable achievements reported over the past three years. Among various biomaterials, synthetic polymers are widely used for fabricating tissue engineering scaffolds due to their excellent biocompatibility and biodegradability. However, their inherent hydrophobicity and suboptimal mechanical properties often limit cell adhesion. The integration of GO or rGO into polymeric scaffolds enables surface modification to overcome these limitations. For instance, Park et al. coated polycaprolactone (PCL) scaffolds with GO to enhance biological performance. This modification increased hydrophilicity, evidenced by a ~ 46% reduction in contact angle, and improved the osteogenic potential of the GO-coated scaffolds (0.5 mg/mL) compared to single PCL scaffold group[76]. Considering the poor interfacial interaction between poly (l-lactic acid) (PLLA) and polyglycolic acid (PGA) which severely restricts the mechanical performance of composite scaffolds, Feng et al. introduced GO to enlarge the contact surface between PLLA and PGA. As a result, the tensile and compressive strengths of the PLLA/PGA scaffolds increased by 44.64% and 69.65%, respectively, and the scaffolds demonstrated good cytocompatibility for cell growth (Figure 3)[77]. However, studies on mechanical behavior have shown that the addition of GO into the PCL may reduce mechanical performance due to the self-agglomeration of GO[78,79]. Nevertheless, the elastic modulus of these scaffolds remains within the range of cancellous/spongy bone, indicating their potential as bone substitutes[80]. In addition, rGO has also been shown to improve cell attachment and osteogenic differentiation of stem cells on PCL scaffolds. Akriti et al. developed a polydopamine (PAD)-rGO reinforced poly-lactic-acid (PLA) scaffold, in which rGO enhanced scaffold hydrophilicity without altering its mechanical properties. Notably, this PLA/GO scaffold exhibited excellent angiogenic and osteogenic capabilities both in vitro and in vivo, representing a promising functional platform for BTE[81-84]. Recent research has also explored the design 3D-printed polyether ether ketone (PEEK) scaffolds. Oladapo et al. fabricated PEEK-rGO-cHAp composite scaffolds via FDM, which showed significant improvements in mechanical strength and biological performance[85-87]. Despite the demonstrated improvements in biological properties and mechanical behavior of polymer scaffolds modified with graphene or its derivatives, a study investigating long-term implantation of graphene-coated polymeric scaffolds reported aberrant electroencephalogram signals and early signs of aging in animal models. These findings suggest that the comprehensive biosafety of G/GO/rGO-integrated polymer scaffolds still requires further evaluation before clinical translation[88].
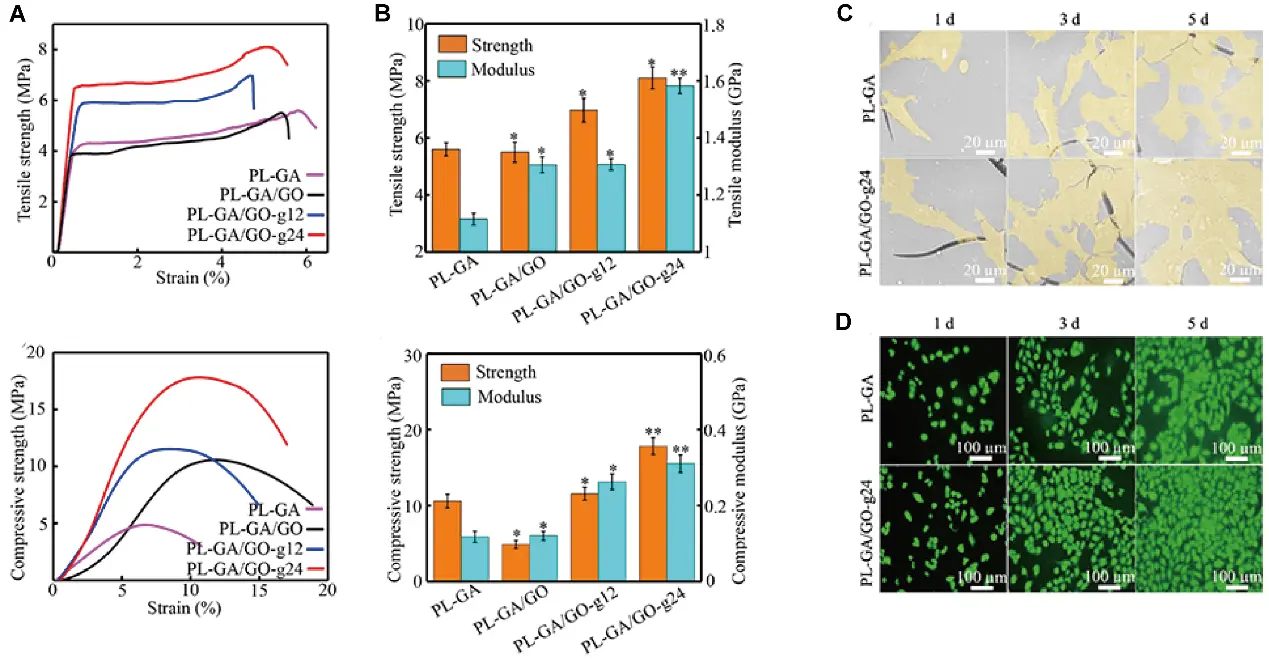
Figure 3. (A) Tensile stress-strain curves and compressive stress strain curves of scaffolds; (B) tensile strength, compressive strength and modulus; (C) morphologies of MG63 cells grew on scaffolds after culturing for different times in vitro; (D) fluorescence images of MG63 cells after culturing for different times. Republished with permission from[77].
Hydrogel-based scaffolds, as 3D scaffolds, have been widely employed in tissue engineering due to their structural similarity to the natural extracellular matrix, as well as their excellent stretchability, flexibility, and biocompatibility. However, their inherently poor mechanical properties limit their application in BTE[89]. On this condition, graphene and its derivatives have been incorporated into hydrogel scaffolds to create multifunctional platforms for promoting bone defect repair. Lai and colleagues developed a GO/HA/CS composite hydrogel scaffold with varying concentration of GO (0.1%/0.25%/0.5%/1%). Their findings demonstrated that increasing the GO concentration improved mechanical properties such as water swelling rate, porosity, and elastic modulus. Notably, the 0.25% GO/HA/CS scaffold exhibited excellent biological performance and biocompatibility with osteoblasts[90]. In both studies, sodium alginate(SA)-based hydrogel scaffolds were modified using magnetic graphene oxide (MGO) and dithiothreitol-modified graphene oxide (DGO) respectively. The incorporation of MGO with an SA-based scaffold not only promotes bone regeneration, but also possesses a magneto-thermal function for bone tumor treatment, and the doping of DGO facilitates cell proliferation while simultaneously offering mechanical support via scaffolds[91,92]. In addition to the above research, GO-doped gellan gum/SF scaffolds with dual functionality have been reported. These scaffolds combine capabilities for bone tumor treatment and tissue regeneration, offering a promising strategy for repairing bone defects following tumor resection[93,94].
In addition to enhancing mechanical performance and promoting osteogenesis when incorporated into polymeric or hydrogel scaffolds, graphene and its derivatives can also be covalently or non-covalently integrated with other biomaterials to further enhance their osteoinductive capabilities. Among these, metals have attracted significant attention in BTE due to their favorable physicochemical properties. Functional groups on the surfaces of graphene-based materials can serve as nucleation sites for anchoring metal atoms, thereby preventing their excessive release and improving cell adhesion, proliferation, and osteogenic differention[95,96]. For example, zinc (Zn), a metallic element with intrinsic antimicrobial properties, also plays a positive role in the proliferation and differentiation of osteoblasts. Khan et al. developed a series of polymeric nanocomposite scaffolds (Zn@rGO-HAp), categorized as AZG-1 through AZG-4 according to increasing Zn content. Each group exhibited enhanced antibacterial and anticancer activities with rising Znlevels. Notably, AZG-4 showed excellent promotion of cell viability and proliferation. Additionally, the combined incorporation of Zn and rGO nanosheets positively influenced the mechanical strength, hydrophilicity, and in vitro degradability of the scaffolds[97,98]. Fani et al. used selenium (Se) and GO to decorate chitosan-alginate polyelectrolyte complex 3D porous scaffolds, in which the simultaneous introduction of GO and Se increased pore density, water absorption, and in vitro biomineralization[99]. Farzin et al. synthesized a zirconia (ZrO2) and rGO-based nanocomposite powder, which significantly enhanced the compressive strength of the scaffold without inducing notable cytotoxicity[100]. In a recent study, GO/Cu-incorporated 3D scaffolds demonstrated strong antibacterial, osteogenic, and angiogenic effects in both a subcutaneous implantation rat model and a bacteria-contaminated bone defect rabbit model. These effects were attributed to the synergistic action of Cu and GO, indicating their potential for treating bacteria- infected bone defects[101].
Benefiting from high specific surface area and abundant functional groups, GO and rGO can readily bind to drug molecules through π-π stacking interactions, making them effective drug delivery carrier in 3D-printed scaffolds. As immunomodulation has become an emerging focus in bone regeneration, researchers have employed 2D GO-BP hetero-nanostructures to enhance the loading capacity of IL-4 cytokines within 3D-scaffolds. These scaffolds enabled the sustained release of IL-4, promoting the polarization of macrophages toward the pro-regenerative M2 phenotype. The synergistic effect of phosphate ion release and IL-4-mediated osteo-immunomodulation ultimately enhanced neovascularization and osteogenesis at bone defect sites[102]. Recent studies have shown that the encapsulation of curcumin in GO-reinforced scaffolds significantly increases drug loading capacity and prolongs release duration under acidic conditions. For instance, curcumin release was sustained over 14 days with approximately 30% release efficiency, and this sustained release synergistically promoted osteogenesis[94,103]. Zhao et al. developed a novel 3D composite scaffold composed of Sr-HAP and rGO, loaded with alendronate sodium (ALN), a common drug used to treat osteoporosis. The stable and prolonged release of ALN was achieved through primary amine group interactions with rGO, as well as hydrogen bonding and Ca-O coordination with Sr-HAP[104]. In the study of the prevention of infection associated with bone transplantation, Zhang et al. fabricated a scaffold composed of TCP/poly(lactic-co-glycolic) acid (PLGA), which incorporated GO nanosheets loaded with the antibacterial chlorhexidine (CHX) and the osteogenic peptide p24. The dual-stage release of CHX and p24 endowed the scaffold with both antibacterial and osteogenic functionalities, offering a promising platform for BTE[105].
3.2 Black phosphorous
As an iconic single-elemental 2D material, BP exhibits a unique atomic structure and exceptional intrinsic properties. Structurally, each phosphorus atom is covalently bonded to three adjacent phosphorus atoms, forming puckered, honeycomb-like layers with exposed lone pair electrons[106]. Such structural characteristics bring BP a large specific surface area that could be used as a drug delivery carrier, but at the same time, the exposed lone pair electrons of phosphorus atoms are readily reactive to oxygen and water, resulting in an instability condition[66]. From a biological perspective, phosphorus is an essential element in the human body and a key component of bone tissue. BP can degraded into phosphate ions, which facilitate local biomineralization by capturing free Ca2+, thereby enhancing the bone repair microenvironment[107]. In addition, due to its excellent photothermal conversion efficiency, BP is regarded as a promising agent for PTT and PDT in antibacterial and antitumor applications, particularly under near-infrared light following bone tumor resection[108].
Based on the above characteristics, appropriate surface modification of BP and its integration with 3D-printed scaffolds have attracted widespread attention in the field of BTE. In a recent study, researchers focusing on implant-related infections after bone surgery proposed a multifunctional bone implant (ZnL2-BPs@HAP), in which BPs was coordinated with a zinc sulfonate ligand (ZnL2) through the interaction between the lone pair electrons of phosphorus and the electron-empty orbital of Zn. This coordination ability was further enhanced by the electrophilic effect of the benzene sulfonic ester. Such a combination exhibited enhanced antibacterial properties, eliminating approximately 91% of S. aureus and 94 % of E. coli eliminated under near-infrared (NIR), while both in vitro and in vivo experiments further confirmed the pro-osteogenic ability of the composite scaffold[109]. Deng and partners constructed BP@AgNPs nanohybrids by decorating silver nanoparticles (AgNPs) onto BPs, and then introduced them into a PLLA scaffold. The broad spectrum bactericidal activity of AgNPs enhanced the synergistic antibacterial property of the composite scaffold. Meanwhile, the scaffold exhibited excellent cytocompatibility, providing a promising and effective photocatalytic antibacterial scaffold for repairing implant-associated bone infections[110]. In another study, researchers developed a chloroplast-inspired 3D CS/PCL/BP/PDA@Ag scaffold, which exhibited excellent self-defensive properties and photothermal stability, and could be used to achieve stronger antibacterial activity and photothermal-induced osteogenesis both in vitro and in vivo (Figure 4)[111].
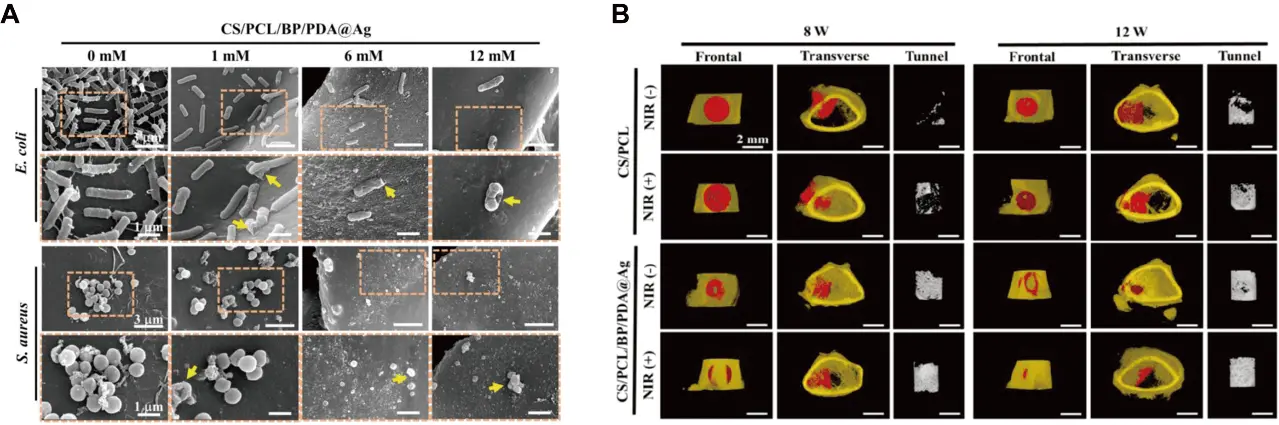
Figure 4. (A) SEM images of E. coli and S. aureus cultured on the scaffolds under different magnification; (B) frontal and transverse views of the 3D-reconstructed images with Micro-CT analysis. Republished with permission from[111]. SEM: scanning electron microscope; Micro-CT: micro-computed tomography; CS: chitosan; PCL: polycaprolactone; BP: black phosphorus; PDA: polydopamine; NIR: near-infrared.
In the field of the integration of BPs with polymeric scaffolds, many new achievements have been reported. For instance, Li et al. proposed a Ca2+ -supplying BP-based PLLA scaffold, in which BP nanosheets not only provided excellent photothermal performance but also served as a P source for bone regeneration through biomineralization. HA-SiO2 nanoparticles also contributed essential trace elements (Ca and Si) for cellular osteogenesis and facilitated new bone formation[112]. In another study, Wang et al. modified BP with poly-dopamine(BP@PDA) to improve its stability, and then integrated it into the PLLA scaffold. The introduction of BP@PDA not only enhanced the mechanical properties but also improved the cytocompatibility of the scaffold[113]. In addition to PDA, CS was used to decorate BP in a 3D-printed PEEK bone scaffold, which effectively protected BP from rapid degradation and promoted the continuous release of PO43- for bone regeneration. Moreover, the composite PEEK scaffold also exhibited excellent antibacterial properties and osteosarcoma ablation in both in vitro and in vivo studies[114]. In a recent study, a multifunctional photothermal scaffold was developed in which bone morphogenetic protein-2 (BMP-2)/PLGA loaded microspheres were synthesized and incorporated into the scaffold. This system promoted osteogenesis by enabling long-term controlled release of BMP-2 and upregulation of heat shock protein expression under NIR irradiation. In addition, PTT-related antibacterial effects are instructive to prevent clinical bacterial infections[115].
Hydrogel-based scaffolds integrated with BP have also achieved notable advancements in the field of BTE. Xu et al. proposed a gelatin-methacryloyl (Gelma) hydrogel composed of BP nanosheets and deferoxamine (DFO) for repairing bone defect, in which the controllable degradation of BP provided phosphorus ions for in-situ mineralization, while DFO enhanced the expression of vascular endothelial growth factor (VEGF) to promote angiogenesis in vitro and in vivo[116]. In another study, researchers developed the dynamic DNA hydrogel incorporating BP nanosheets loading with VEGF. The non-covalent interaction between VEGF and BPNSs enabled sustained VEGF release, which synergistically promoted osteogenesis and angiogenesis, thereby enhancing bone regeneration[117]. In a study using a coaxial microfluidic printing strategy, Wang et al. fabricated hydrogel scaffolds composed of alginate and N-isopropyl acrylamide incorporating BP nanosheets. These scaffolds exhibited reversible shrinkage and swelling behavior due to the NIR heating capability of BP and the temperature- responsive volume phase transition of the hydrogel. The composite scaffolds promoted osteogenic differentiation of bone MSCs, as well as facilitated angiogenesis and the ingrowth of vascular endothelial cells into the scaffolds[118].
TMDs are a class of 2D materials with the general form MX2, where M represents a transition metal from groups 4 to 7 (e.g., Mo, W, Ta, Nb, Re, and Mn), and X refers to a chalcogen element (S, Se, Te)[119]. Similar to graphite, TMDs possess a layered structure held together by van der Waals forces. Each monolayer typically exhibits a low thickness of approximately 0.6-0.7 nm and a large specific surface area[120]. In addition, TMDs exhibit low cytotoxicity, good cytocompatibility, and the ability to promote osteogenic differentiation, which supports their potential applications in biological and biomedical fields, particularly in BTE.
Among the family of TMDs, MoS2 is one of the most extensively studied materials due to its robustness, ease of surface modification, and excellent NIR photothermal conversion efficiency[121]. In a recent study, a novel bioactive glass (BGs)-based 3D-printed scaffold was fabricated for local tumor ablation and bone tissue regeneration, in which MoS2-PLGA film was fixed onto the surface of the BG scaffolds. The incorporated MoS2 nanosheets provided excellent photothermal stability under 808 nm NIR irradiation for PTT. In addition, as an essential trace element, Mo can contribute to coordinated local bone repair by participating in the synthesis of several important enzymes[20]. Benefiting from the ideal mechanical characteristics and efficient photothermal response of MoS2, Dai et al. modified a PEEK scaffold with MoS2 nanosheets and HA nanoparticles to construct a dual-functional scaffold exhibiting both potent PTT effects and osteogenic induction. This system achieved a balance between tumor ablation and bone regeneration[122]. Under normal conditions, monolayered MoS2 nanosheets tend to self-stack and aggregate, limiting their practical application. To overcome this issue, Qi et al. introduced polyaniline (PANI) to MoS2 nanosheets via in situ oxidative polymerization, forming strong coordination bonds between the nitrogen atoms of PANI and the molybdenum centers. The resulting PANI-MoS2 composite was integrated into PVDF scaffolds, which enhanced the mechanical strength, hydrophilicity, and piezoelectric properties of the scaffolds. The improved electrical signals were shown to activate protein kinase C and mitogen-activated protein kinase signaling pathways, thereby promoting stem cell proliferation, differentiation, and osteogenic gene transcription[123]. In another study, researchers fabricated a co-dispersion nanosheets system, in which MoS2 nanosheets were in situ synthesized on the surface of GO nanosheets using a cetyltrimethylammonium bromide (CTAB)-assisted hydrothermal process to improve dispersion with a PLLA scaffold (Figure 5). The PLLA scaffold loaded with 1.5 wt% GO/MoS2 demonstrated enhanced tensile strength and modulus, as well as favorable cytocompatibility, showing potential for application in bone implants[124]. In the context of implant-related infections following bone tumor surgery, our group designed a composite PGA scaffold incorporating an Ag@PMoS2 nanosystem via in situ reduction. The excellent NIR photothermal properties of MoS2, combined with the natural antibacterial activity of silver ion (Ag+), acted synergistically to eliminate bacteria and induce apoptosis in tumor cells[125].
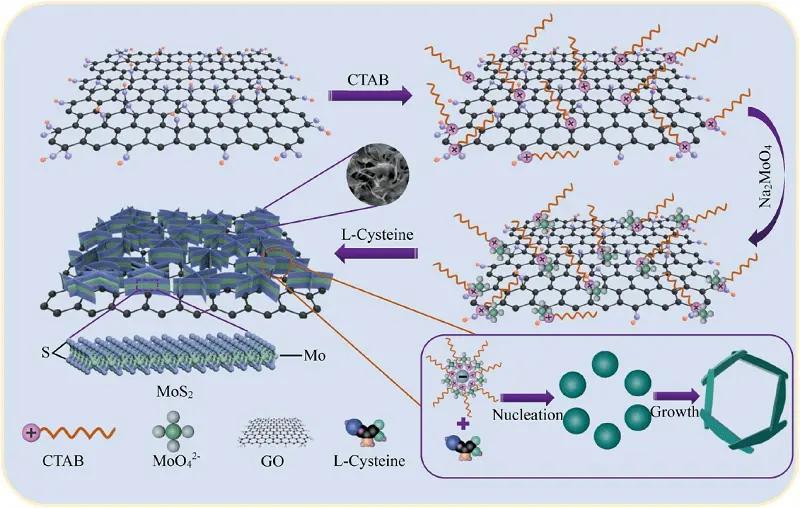
Figure 5. The mechanism diagram of the MoS2 nanosheets in situ grown on both sides of GO nanosheets via CTAB-assisted hydrothermal process. Republished with permission from[124]. GO: graphene oxide; CTAB: hexadecyl trimethyl ammonium bromide.
In addition to MoS2, recent studies focused on WS2 and MoSe2 nanosheets have been reported in the field of 3D-printed scaffolds. Similar to MoS2, WS2 exhibits excellent biocompatibility and physicochemical properties. Geng et al. constructed a multifunctional 3D p-CQD/WS2/n-CQD/GelMA hydrogel scaffold, in which the introduction of WS2 could achieve the controlled release of p-CQDs and enhance the mechanical property of hydrogel scaffold, this nanocomposite scaffold possesses the excellent broad-spectrum antibacterial ability and osteogenic activity, which realized almost fully repair of infectious bone defect 60 days after implantation[126]. In another study, researchers introduced MoSe2 nanocrystals into bioactive bredigite scaffolds, thereby combining photothermal properties with osteogenic activities. This approach may offer a promising strategy for the treatment of bone defects resulting from tumor resection[127].
3.4 Mxenes
As a new class of 2D NMs, Mxenes comprise a large group of 2D transition metal carbides, carbonitrides, and nitrides. They possess a range of physicochemical properties, including a large specific surface area suitable for drug loading, excellent photothermal conversion efficiency for antibacterial and tumor-killing applications, outstanding biocompatibility, and the ability to promote osteogenesis by providing Ca2+ binding sites. These characteristics highlight their potential for application in bone tissue regeneration[128,129].
Among the family of Mxenes, the first reported material is Ti3C2, which exhibits excellent biomedical properties. Notably, during its interaction with water and oxygen, Ti3C2 can degrade into titanium (Ti)-based species that promote new bone formation, making it a promising candidate for applications in BTE. Recent studies have reported the integration of Ti3C2 into 3D-printed scaffolds. For instance, Mi et al. fabricated a Ti3C2-modified HA/SA composite scaffold, in which the inclusion of Ti3C2 improved surface roughness and created a porous, interconnected structure, facilitating the diffusion of H2O, O2, and bioactive substances[130]. In the study of the hydrogel-based scaffold, researchers developed a personalized MXene-based bifunctional construct (β-TCP/Alg/Ti3C2), where Ti3C2 contributed excellent photothermal performance for G+/G- bacteria killing, while also promoting the proliferation and differentiation of rBMSCs[131]. In addition to its antibacterial properties, Ti3C2 has also been proven to be an effective photothermal agent for tumor ablation under NIR irradiation. Li et al. constructed a Ti3C2-modified collagen/silk/hydroxyapatite composite scaffold for killing residual cancer cells, achieving a necrosis rate of approximately 91%, while simultaneously repairing bone defects post-surgery. This provides a potential platform for treating bone defects associated with bacterial infection or tumor resection[132]. In investigation of the molecular mechanisms underlying bone regeneration by MXene-based scaffolds, Xu et al. sequenced the differentially expressed RNAs in osteoblasts cultured on the scaffolds for 14 days. KEGG pathway classification revealed that these RNAs were mainly involved in the Wnt signaling pathway. Additionally, upregulation of the Titin gene was shown to regulate cell proliferation by activating the Wnt/β-catenin pathway, suggesting that Ti-based Mxenes may facilitate osteogenesis through the Wnt/β-catenin signaling pathway and calcium-binding proteins[133].
As a novel type of 2D Mxenes, Nb2C has attracted widespread attention due to its excellent photoresponsive property in the second NIR-II biological window, making it potentially applicable for the treatment of tumor-induced bone defects[134]. Chen et al. integrated Nb2C nanosheets with a 3D-printed BG scaffold. This composite scaffold enabled deeper tissue penetration under NIR-II irradiation for osteosarcoma therapy. In addition, the presence of Nb imparted pro-angiogenic properties to the scaffold, thereby facilitating bone defect regeneration (Figure 6)[135]. In the context of breast cancer-related bone metastasis, Chen et al. further designed an Nb2C-modified BG scaffold loaded with the immune adjuvant R837. This system facilitated checkpoint blockade immunotherapy to eliminate primary tumors, activate the immune response, suppress tumor metastasis, and thereby prevent tumor recurrence[136]. Besides the above research, this lab also reported a porous silica-coated 2D Nb2C and S-nitrosothiol (NO donor) integrated 3D-printed BG scaffold(named MBS). The synergistic effect of NO gas and Nb2C enhanced the antitumor effect under NIR irradiation. Moreover, the sustained release of NO and Nb-based species jointly promoted bone regeneration, providing a promising multifunctional platform for the treatment of bone tumors[137].
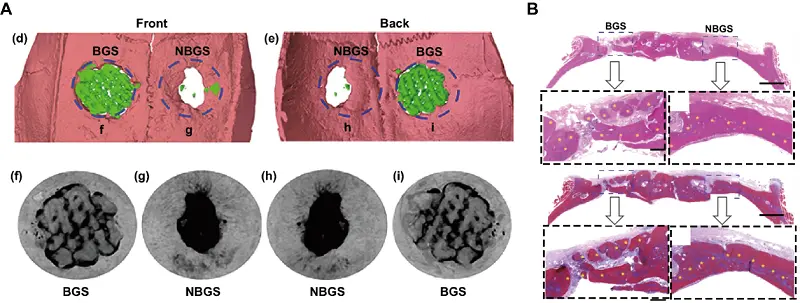
Figure 6. (A) 3D reconstruction of Micro-CT scanning was obtained to assess the defect and bone regeneration with different scaffolds; (B) H&E and Masson trichrome staining of the cranial defect implanted with BGS/NBGS at week 24. Republished with permission from[135]. Micro-CT: microcomputed tomography; BGS: bioactive glass scaffolds; NBGS: 3D-printed bone-mimetic scaffolds.
3.5 LAP
LAP is a synthetic lithium-magnesium-sodium silicate 2D NM that possesses unique characteristics such as good biocompatibility and biodegradability, a large surface area, enhanced mechanical strength and hydrophilicity, as well as a strong adsorption capacity for binding proteins and other biomolecules. These properties make LAP a promising biomaterial platform for the sustained delivery of various regenerative molecules or drugs[138]. In addition, the biodegradation of LAP can release inorganic ions (Mg2+, Si(OH)4, and Li), which have been shown to exert positive effects on new bone regeneration[17,139]. Cebe et al. fabricated a methacrylate chitosan (MAC)-based 3D scaffold integrated with LAP, which exhibited improved solubility, mechanical, and morphological properties. Furthermore, the increased expression of collagen synthesis promoted mineral formation due to the combined effects of MAC and LAP[140]. Similar results were reported by Xu and collaborators, who confirmed the positive effects of 3D-printed PCL/LAP scaffold on the osteogenesis and angiogenesis in bone defect models. However, the prolonged degradation period of the PCL/LAP scaffold, still present three months after implantation, might hinder in situ bone regeneration and warrants further investigation [141]. Subsequent research demonstrated that the addition of LAP significantly improved the loading capacity of PCL scaffolds for growth factors, which facilitated vascularization and enhanced bone regeneration in a rat femoral condyle defect model[142]. To explore the effects of different LAP contents on bone repair, researchers introduced varied LAP concentrations into a 3D-printed PLGA/β-TCP composite scaffold (PTL). The mechanical properties and degradation rate of the scaffold increased with LAP content, and the scaffold containing 20 % LAP exhibited the best performance in promoting angiogenesis and osteogenesis of rBMECs[143]. In the field of LAP-doped hydrogel-based scaffolds, Dong et al. developed a nanocomposite hydrogel composed of 15% GelMA and 8% LAP. In this system, LAP significantly enhanced shape fidelity and mechanical strength. The resulting hydrogel scaffold demonstrated good printability and osteogenic property for bone regeneration[144]. The specific osteogenic mechanism of the LAP-containing hydrogel scaffolds was further investigated by Miao et al., RNA sequencing revealed that activation of PI3K/AKT signaling pathway in BMSCs enhanced extracellular matrix formation and mineralization, which could be suppressed by a PI3K inhibitor[138]. These findings suggest that 3D LAP-based hydrogel scaffolds may serve as promising substitutes for the reconstruction of bone defects.
3.6 MOF
As a functional 2D NM, MOF are typically composed of metal ions (e.g., Zn, Mg, and Cu) regularly coordinated with organic ligands. MOFs have attracted widespread attention due to their unique structure and excellent physicochemical properties, including high porosity and large specific surface area[21]. The porous structure allows for efficient drug delivery, while the biodegradation of MOF can release metal ions that enhance bone regeneration and exert antibacterial effects. Therefore, the application of MOF-integrated 3D-printed scaffolds represents a promising strategy for bone defect repair. In a recent study, researchers fabricated a 3D-printed PCL scaffold composited with nanoZIF-8 and dicalcium phosphate dehydrate. This scaffold exhibited a biomimetic structure with suitable mechanical properties and biocompatibility, while the controlled release of Ca and Zn ions facilitated osteogenesis both in vitro and in vivo[145]. Inspired by the reports of zinc-cobalt bimetallic MOF exhibiting excellent ROS scavenging ability, Shu et al. developed a β-TCP scaffold functionalized with Zn/Co-MOF for treating osteoarthritis-related defects. This scaffold not only protected cells from oxidative damage by eliminating ROS, but also stimulated targeted differentiation by releasing multiple bioactive ions[146]. In the application of drug delivery, Jiang et al. employed pZIF-8 nanoMOFs as drug nanocarriers to encapsulate both BMP-2 and cisplatin. These MOF nanocarriers were then integrated into a 3D-printed PDA@HA gelatin scaffold. The system enabled on-demand release of cisplatin on to inhibit tumor growth, along with sustained release of BMP-2 to promote osteogenesis[147]. In another study, Wang et al. developed a multifunctional 3D-printed Ti6Al4V scaffold incorporated with Mg-MOF-74, which served as a controlled-release platform for the long-term delivery of icariin. The sustained release of both icariin and Mg2+ significantly improved osteointegration between the scaffold and osteoporotic bone, offering a potential therapeutic strategy for elderly patients with osteoporosis[148].
3.7 Layered double hydroxide
LDH, also known as synthetic anionic clays, have the formula [M2+(1-x)M3+x(OH)2]x+[(An−)x/nzH2O]x-. They consist of positively charged metal hydroxide layers and exchangeable interlayer anions, which endow them with potential for drug delivery applications[149]. In addition, the positive surface charge of LDHs enables electrostatic interaction with negatively charged cell membranes, facilitating cell adhesion. Moreover, the released Mg2+ and Al3+ ions play an active role in the osteogenesis and angiogenesis of BMSCs[150]. In the investigation of drug-loading capabilities of LDH-integrated 3D-printed scaffolds, Belgheisi et al. fabricated pamidronate (PAM)-loaded LDH/PCL scaffolds for BTE applications. In this system, LDHs ensured the long-term and stable release of PAM through an ion-exchange mechanism between the intercalated PAM molecules and the exchangeable interlayer anions[15]. In another study, researchers introduced ciprofloxacin and gentamicin into LDH-filled scaffold. The sustained release of these antibiotics relied on ion-exchange reactions or pH-sensitive mechanisms, thereby providing dual functionality for both bone regeneration and infection prevention[151]. In the field of 3D bioprinting for tissue engineering, Ayça'lab reported a nanocomposite hydrogel bioink composed of GelMA and LDH nanoparticles, laden with hBMSCs. The scaffold pore size significantly increased with higher LDH content, and immunofluorescence staining demonstrated that LDHs enhanced both the viability and osteogenesis of hBMSCs, indicating a positive effect on bone defect repair[152,153].
4. Conclusion and Prospects
This review has summarized the recent progress in the integration of 2D NMs with 3D printed scaffolds for applications in BTE. Owing to their intrinsic characteristics, such as large surface area, superior electrical and optical properties, and abundant surface-active sites, 2D NMs have been rapidly developed for use in drug delivery, photo-thermal/photodynamic therapy, biosensing, and diagnostic imaging. Furthermore, many 2D NMs have demonstrated excellent mechanical strength and pro-osteogenic capabilities. By fully leveraging these properties, 3D-printed scaffolds can be endowed with multifunctionality, thereby enhancing their performance in BTE applications. The primary contributions of 2D NMs in this context can be summarized as follows: 1. Reinforced mechanical properties; 2. Photothermal effect for bone-tumor killing; 3. Enhanced antibacterial abilities; 4. Intrinsic induction of osteogenesis; 5. Improved drug delivery capacity. The exploration of combining different types of 2D NMs to achieve synergetic effects may represent a promising strategy for optimizing therapeutic outcomes and further expanding the applications of 2D NMs.
Nevertheless, several issues and challenges must be addressed before the clinical translation of 2D NMs. First, although the majority of 2D NMs exhibit good biocompatibility, their potential toxicity remains a concern. For instance, the agglomeration of 2D NMs can lead to inhomogeneous printing inks, adversely affecting scaffold performance. In addition, weak interfacial bonding between nanomaterials and matrix materials may cause delamination, which can induce significant oxidative stress and mechanical disruption to the cell membrane — factors that play a critical role in cell damage and associated toxicity[65]. Moreover, the cytotoxicity of non-degradable 2D NMs has been shown to be time- and concentration-dependent. Especially in the case of long-term scaffold implantation, the local accumulation of such materials may lead to chronic toxicity, including chronic inflammation and granuloma formation[154]. For degradable 2D NMs, controlling degradation controlling. For example, BP degrades into phosphate, which can promote the biomineralization of BMSCs in culture. However, the degradation rate of unmodified BP is excessively rapid, releasing large amounts of H ions and PO4 ions in a short period, leading to a local acidic environment that is unfavorable for cell growth[155]. To improve the stability and biosafety of 2D NMs, organic polymers such as PEG, its derivatives, and PDA have been employed[113,70]. Nevertheless, considering the potential risks of long-term in vivo biosafety and biodegradability, the development of new functionalization strategies remains essential for future research.
Secondly, many 2D NMs exhibit excellent photothermal conversion efficiency, making them suitable for the treatment of bone defects caused by infections or tumor resection when integrated with 3D-printed scaffolds. Current studies primarily rely on high temperature (> 55 °C) and ROS to kill tumor cells and bacteria. However, this approach may cause irreversible thermal damage to surrounding healthy tissues, which can adversely affect[156,157]. Notably, moderate photothermal stimulation at 40-42 °C has been shown to enhance osteogenesis[158]. Therefore, whether future strategies can increase the photothermal sensitivity of bacteria and tumor cells to effective antibacterial and antitumor therapy at lower temperature (< 50 °C) with minimal side effects remains an important area of investigation. Immune modulation has emerged as a promising strategy to promote bone regeneration by regulating immune cell behavior and cytokine secretion. In recent years, the design of biomaterials with immunomodulatory functions has become a research hotspot. For instance, nanomaterials and hydrogels can be engineered to deliver immunomodulatory drugs or growth factors, enabling sustained release at the bone injury site to continuously modulate immune response and promote tissue regeneration. In addition, the physical properties of biomaterials, such as surface charge and roughness, can influence immune cell adhesion, proliferation, and differentiation, thereby indirectly affecting the bone healing process. However, immune modulation strategies still face some challenges in clinical translation, particularly regarding the precision and safety of immune regulation, which require further in-depth research and exploration.
Furthermore, as the implantation of scaffolds often results in permanent outcome, it's essential to develop appropriate nanocomposite implants tailored to the specific requirements of bone defects in different anatomical locations. At the same time, the long-term biosafety of 2D NM-based scaffolds must be thoroughly evaluated in vivo over extended periods. In addition, scaffold degradation typically occurs in parallel with the regeneration of bone tissue, blood vessels, and nerves. While significant progress has been made in promoting the osteogenic differentiation of BMSCs using 2D NM-based scaffolds, their potential roles in neurogenic and angiogenic differentiation should not be overlooked. With the growing variety of available 2D NMs, the incorporation of novel types of 2D NMs into 3D-printed scaffolds is expected to expand. Moreover, the strategic combination of different 2D NMs to achieve synergistic effects represents a promising trend in BTE. Future research should address those critical issues to support the clinical translation of 2D NM-based scaffolds.
Authors contributions
Peng S: Conceived the idea for the article, revised the manuscript.
Niu Z: Drafted the manuscript.
He T: Revised the manuscript, conducted the literature search.
Conflicts of interest
The authors declare no competing interests.
Ethical approval
Not applicable.
Consent to participate
Not applicable.
Consent for publication
Not applicable.
Availability of data and materials
Not applicable.
Funding
This study was supported by the following funds: (1) The National Natural Science Foundation of China (No. 81871498, 82072084, 51935014, 52275393,52275395); (2) JiangXi Provincial Natural Science Foundation of China (20224ACB204013); (3) Innovation for graduate students of Central South University (2024ZZTS0511).
Copyright
© The Author(s) 2025.
References
-
1. Moraschini V, de Almeida DCF, Calasans-Maia MD, Cavalcante Kischinhevsky, Louro RS, Granjeiro JM. Immunological response of allogeneic bone grafting: A systematic review of prospective studies. J Oral Pathol Med. 2020;49 (5):395-403.
[DOI] -
2. Titsinides S, Agrogiannis G, Karatzas T. Bone grafting materials in dentoalveolar reconstruction: a comprehensive review. Jpn Dent Sci Rev. 2019;55 (1):26-32.
[DOI] -
3. Wei H, Cui J, Lin K, Xie J, Wang X. Recent advances in smart stimuli-responsive biomaterials for bone therapeutics and regeneration. Bone Res. 2022;10(1):17.
[DOI] -
4. Place ES, Evans ND, Stevens MM. Complexity in biomaterials for tissue engineering. Nature Mater. 2009;8(6):457-470.
[DOI] -
5. Wang C, Huang W, Zhou Y, He L, He Z, Chen Z, et al. 3D printing of bone tissue engineering scaffolds. Bioact Mater. 2020;5, (1):82-91.
[DOI] -
6. Shuai C, Zhao Y, Deng Y, Gao C. Heterogeneous grain structure in biodegradable Zn prepared via mechanical alloying and laser powder bed fusion for strength-plasticity synergy. Virt Phys Prototyp. 2024;19(1):e2317780.
[DOI] -
7. Yuan X, Zhu W, Yang Z, He N, Chen F, et al. Recent Advances in 3D printing of smart scaffolds for bone tissue engineering and regeneration. Adv Mater. 2024;36(34):2403641.
[DOI] -
8. Murphy SV, Atala A. 3D bioprinting of tissues and organs. Nat Biotechnol. 2014;32(8):773-785.
[DOI] -
9. Culmone C, Smit G, Breedveld P. Additive manufacturing of medical instruments: a state-of-the-art review. Addit Manuf. 2019;27:461-473.
[DOI] -
10. Collins MN, Ren G, Young K, Pina S, Reis RL, Oliveira JM. Scaffold Fabrication Technologies and Structure/Function Properties in Bone Tissue Engineering. Adv Funct Mater. 2021;31:(21).
[DOI] -
11. Hassan M, Dave K, Chandrawati R, Dehghani F, Gomes VG. 3D printing of biopolymer nanocomposites for tissue engineering: nNanomaterials, processing and structure-function relation. Eur Polym J. 2019;121:109340.
[DOI] -
12. Vithani K, Goyanes A, Jannin V, Basit AW, Gaisford S, Boyd BJ. An overview of 3D printing technologies for soft materials and potential opportunities for lipid-based drug delivery systems. Pharm Res. 2019;36:4.
[DOI] -
13. Qin L, Yang S, Zhao C, Yang J, Li F, Xu Z, et al. Prospects and challenges for the application of tissue engineering technologies in the treatment of bone infections. Bone Res. 2024;12:28.
[DOI] -
14. Novoselov KS, Geim AK, Morozov SV, Jiang D, Zhang Y, Dubonos SV, et al. Electric field effect in atomically thin carbon films. Science. 2004;306 (5696):666-669.
[DOI] -
15. Belgheisi G, Nazarpak MH, Solati-Hashjin M. Fabrication and evaluation of combined 3D printed/pamidronate-layered double hydroxides enriched electrospun scaffolds for bone tissue engineering applications. Appl Clay Sci. 2022;225:106538.
[DOI] -
16. Ding L, Wei Y, Wang Y, Chen H, Caro J, Wang H. A two-dimensional lamellar membrane: MXene nanosheet stacks. Angew Chem Int Ed. 2017;56(7):1825-1829.
[DOI] -
17. Gaharwar AK, Mihaila SM, Swami A, Patel A, Sant S, Reis RL, et al. Bioactive silicate nanoplatelets for osteogenic differentiation of human mesenchymal stem cells. Adv Mater. 2013;25 (24):3329-3336.
[DOI] -
18. Hanlon D, Backes C, Doherty E, Cucinotta CS, Berner NC, Boland C, et al. Liquid exfoliation of solvent-stabilized few-layer black phosphorus for applications beyond electronics. Nat Commun. 2015;6:8563.
[DOI] -
19. Stoller MD, Park SJ, Zhu YW, An JH, Ruoff RS. Graphene-Based Ultracapacitors. Nano Lett. 2008;8(10):3498-3502.
[DOI] -
20. Wang H, Zeng X, Pang L, Wang H, Lin B, Deng Z, et al. Integrative treatment of anti-tumor/bone repair by combination of MoS2 nanosheets with 3D printed bioactive borosilicate glass scaffolds. Chem Eng J. 2020;396:125081.
[DOI] -
21. Zhong L, Chen J, Ma Z, Feng H, Chen S, Cai H, et al. 3D printing of metal-organic framework incorporated porous scaffolds to promote osteogenic differentiation and bone regeneration. Nanoscale. 2020;12(48):24437-24449.
[DOI] -
22. Jeon IY, Shin YR, Sohn GJ, Choi HJ, Bae SY, Mahmood J, et al. Edge-carboxylated graphene nanosheets via ball milling. Proc Natl Acad Sci USA. 2012;109 (15):5588-5593.
[DOI] -
23. Liu N, Kim P, Kim JH, Ye JH, Kim S, Lee CJ. Large-area atomically thin MoS2 nanosheets prepared using electrochemical exfoliation. Acs Nano. 2014;8(7):6902-6910.
[DOI] -
24. Lu LS, Chen GH, Cheng HY, Chuu CP, Lu KC, Chen CH, et al. Layer-dependent and in-plane anisotropic properties of low-temperature synthesized few-layer PdSe2 single crystals. Acs Nano. 2020;14(4):4963-4972.
[DOI] -
25. Shen J, He Y, Wu J, Gao C, Keyshar K, Zhang X, et al. Liquid phase exfoliation of two-dimensional materials by directly probing and matching surface tension components. Nano Lett. 2015;15(8):5449-5454.
[DOI] -
26. Takahashi Y, Nakayasu Y, Iwase K, Kobayashi H, Honma I. Supercritical hydrothermal synthesis of MoS2 nanosheets with controllable layer number and phase structure. Dalton Trans. 2020;49(27):9377-9384.
[DOI] -
27. Zhou X, Gan L, Zhang Q, Xiong X, Li H, Zhong Z, et al. High performance near-infrared photodetectors based on ultrathin SnS nanobelts grown via physical vapor deposition. J Mater Chem C. 2016;4(11):2111-2116.
[DOI] -
28. Cheng L, Wang X, Gong F, Liu T, Liu Z. 2D Nanomaterials for cancer theranostic applications. Adv Mater. 2020;32:(13):1902333.
-
29. Yang K, Feng L, Shi X, Liu Z. Nano-graphene in biomedicine: theranostic applications. Chem Soc Rev. 2013;42(2):530-547.
[DOI] -
30. Qi F, Liu W, Li Z, Peng S, Chen Y, Tan W, et al. Reactive oxygen species responsive nitric oxide release for enhanced photodynamic antibacterial therapy of scaffolds. ACS Appl Polym Mater. 2024;6(11):6581-6593.
[DOI] -
31. Yang W, Zhou C, He C, Yang Y, Aiyiti W, Xu L, et al. Defect engineering synergistically boosts the catalytic activity of Fe-MoOv for highly efficient breast mesh antitumor therapy. J Colloid Interface Sci. 2025;678(Part A):260-271.
[DOI] -
32. Chen J, Zhou H, Fan Y, Gao G, Ying Y, Li J. 3D printing for bone repair: Coupling infection therapy and defect regeneration. Chem Eng J. 2023;471:144537.
[DOI] -
33. Aidun A, Firoozabady AS, Moharrami M, Ahmadi A, Haghighipour N, Bonakdar S, et al. Graphene oxide incorporated polycaprolactone/chitosan/collagen electrospun scaffold: enhanced osteogenic properties for bone tissue engineering. Artif Organs. 2019;43(10):E264-E281.
[DOI] -
34. Feng L, Liu Z. Graphene in biomedicine: opportunities and challenges. Nanomedicine. 2011;6(2):317-324.
[DOI] -
35. Yang W, Tong Q, He C, He T, Shuai X, Song H, et al. Mechanically propelled ion exchange regulates metal/bioceramic interface characteristics to improve the corrosion resistance of Mg composite for orthopedic applications. Ceram Int. 2024;50(13):23124-23134.
[DOI] -
36. Lee D, Lee B, Park KH, Ryu HJ, Jeon S, Hong SH. Scalable exfoliation process for highly soluble boron nitride nanoplatelets by hydroxide-assisted ball milling. Nano Lett. 2015;15(2):1238-1244.
[DOI] -
37. Wu Z, Fang B, Bonakdarpour A, Sun A, Wilkinson D, Wang D. WS2 nanosheets as a highly efficient electrocatalyst for hydrogen evolution reaction. Appl Catal B. 2012;125:59-66.
[DOI] -
38. Li LH, Chen Y, Behan G, Zhang H, Petravic M, Glushenkov AM. Large-scale mechanical peeling of boron nitride nanosheets by low-energy ball milling. J Mater Chem. 2011;21(32):11862-11866.
[DOI] -
39. Varrla E, Backes C, Paton KR, Harvey A, Gholamvand Z, McCauley J, et al. Large-scale production of size-controlled MoS2 nanosheets by shear exfoliation. Chem Mater. 2015;27(3):1129-1139.
-
40. Bai H, Li C, Shi G. Functional composite materials based on chemically converted graphene. Adv Mater. 2011;23(9):1089-1115.
[DOI] -
41. Zamhuri A, Lim GP, Ma NL, Tee KS, Soon CF. MXene in the lens of biomedical engineering: synthesis, applications and future outlook. BioMed Eng OnLine. 2021;20:33.
[DOI] -
42. Naguib M, Come J, Dyatkin B, Presser V, Taberna PL, Simon P, et al. MXene: a promising transition metal carbide anode for lithium-ion batteries. Electrochem Commun. 2012;16(1):61-64.
[DOI] -
43. Ghidiu M, Lukatskaya MR, Zhao MQ, Gogotsi Y, Barsoum MW. Conductive two-dimensional titanium carbide 'clay' with high volumetric capacitance. Nature. 2014;516(7529):78-81.
[DOI] -
44. Liu FF, Zhou A, Chen J, Jin J, Zhou W, Wang L, et al. Preparation of Ti3C2 and Ti2C MXenes by fluoride salts etching and methane adsorptive properties. Appl Surf Sci. 2017;416:781-789.
[DOI] -
45. Feng A, Yu Y, Wang Y, Jiang F, Yu Y, Mi L, et al. Two-dimensional MXene Ti3C2 produced by exfoliation of Ti3AlC2. Mater Des. 2017;114:161-166.
[DOI] -
46. Jiang K, Ji J, Gong W, Ding L, Li J, Li P, et al. Mechanical cleavage of non-van der Waals structures towards two-dimensional crystals. Nat Synth. 2022;2:58-66.
[DOI] -
47. Xue Y, Zhao G, Yang R, Chu F, Chen J, Wang L, et al. 2D metal-organic framework-based materials for electrocatalytic, photocatalytic and thermocatalytic applications. Nanoscale. 2021;13(7):3911-3936.
[DOI] -
48. Feng P, He R, Yang F, Lin Y, Fan L, Pan H, et al. Photocatalytic antibacterial scaffold for inhibiting bacterial infection: carriers separation, ROS generation and antibacterial action. Sustain Mater Technol. 2025;43:e01205.
[DOI] -
49. Kaur R, Gaur A, Pundir V, Arun K, Bagchi V. Unleashing unprecedented activation of high-valent Ni and Fe charge dynamics in CeF3-NiFe layered double hydroxide heterostructure: demonstrating oxygen evolution reaction at an extremely high current density. J Colloid Interface Sci. 2024;672:736-743.
[DOI] -
50. Yang W, Ji Y, Li L, Ding C, Aiyiti W, Xiong F, et al. Persistent glutathione-depleting MFO@MIL nanoreactors enhance the antitumor efficiency of a skin scaffold. Mater Chem Front. 2025;9:1249-1258.
[DOI] -
51. Yang W, Ding C, Ji Y, He C, Xiong F, Aiyiti W, et al. Self-augmented catabolism mediated by Se/Fe co-doped bioceramics boosts ROS storm for highly efficient antitumor therapy of bone scaffolds. Colloids Surf B Biointerfaces. 2025;248:114477.
[DOI] -
52. Kuriakose S, Jain SK, Tawfik SA, Spencer MJS, Murdoch BJ, Singh M, et al. Monocrystalline antimonene nanosheets via physical vapor deposition. Adv Mater Interfaces. 2020;7(24):2001678.
[DOI] -
53. Qi F, Chen Y, Zheng B, Zhou J, Wang X, Li P, et al. Facile growth of large-area and high-quality few-layer ReS2 by physical vapour deposition. Mater Lett. 2016;184:324-327.
[DOI] -
54. Sun X, An X, Zhang S, Li Z, Zhang J, Wu W, et al. Physical vapor deposition (PVD): a method to fabricate modified g-C3N4 sheets. New J Chem. 2019;43(17):6683-6687.
[DOI] -
55. Wang Y, Li H, Yang T, Zou Z, Qi Z, Ma L, et al. Space-confined physical vapour deposition of high quality ZnTe nanosheets for optoelectronic application. Mater Lett. 2019;238:309-312.
[DOI] -
56. Hong YL, Liu ZB, Wang L, Zhou TY, Ma W, Xu C, et al. Chemical vapor deposition of layered two-dimensional MoSi2N4 materials. Science. 2020;369:670-674.
[DOI] -
57. Marcinek A, Hardwick LJ, Richardson TJ, Song X, Kostecki R. Microwave plasma chemical vapor deposition of nano-structured Sn/C composite thin-film anodes for Li-ion batteries. J Power Sources. 2007;173(2):965-971.
[DOI] -
58. Somani PR, Somani SP, Umeno M. Planer nano-graphenes from camphor by CVD. Chem Phys Lett. 2006;430 (1-3):56-59.
[DOI] -
59. Wu Q, Song YJ. The environmental stability of large-size and single-crystalline antimony flakes grown by chemical vapor deposition on SiO2 substrates. Chem Commun. 2018;54(69):9671-9674.
[DOI] -
60. Lee YH, Zhang XQ, Zhang W, Chang MT, Lin CT, Chang KD, et al. Synthesis of large-area MoS2 atomic layers with chemical vapor deposition. Adv Mater. 2012;24(17):2320-2325.
[DOI] -
61. Shim GW, Yoo K, Seo SB, Shin J, Jung D, Kang IS, et al. Large-area single-layer MoSe2 and its van der Waals heterostructures. Acs Nano. 2014;8(7):6655-6662.
[DOI] -
62. Zhang Y, Zhang Y, Ji Q, Ju J, Yuan H, Shi J, et al. Controlled growth of high-quality monolayer WS2 layers on sapphire and imaging its grain boundary. Acs Nano. 2013;7(10):8963-8971.
[DOI] -
63. Li Z, Wang L, Li Y, Feng Y, Feng W. Carbon-based functional nanomaterials: preparation, properties and applications. Compos Sci Technol. 2019;179:10-40.
[DOI] -
64. Gao MR, Liang JX, Zheng YR, Xu YF, Jiang J, Gao Q, et al. An efficient molybdenum disulfide/cobalt diselenide hybrid catalyst for electrochemical hydrogen generation. Nat Commun. 2015;6:5982.
[DOI] -
65. Akhavan O, Ghaderi E, Akhavan A. Size-dependent genotoxicity of graphene nanoplatelets in human stem cells. Biomaterials. 2012;33(32):8017-8025.
[DOI] -
66. Li Z, Guo T, Hu Y, Qiu Y, Liu Y, Wang H, et al. A highly effective π-π stacking strategy to modify black phosphorus with aromatic molecules for cancer theranostics. ACS Appl Mater. Interfaces. 2019;11(10):9860-9871.
[DOI] -
67. Wu M, Wang B, Hu Q, Wang L, Zhou A. The synthesis process and thermal stability of V2C MXene. Materials. 2018;11(11):2112.
[DOI] -
68. Depan D, Girase B, Shah JS, Misra RDK. Structure-process-property relationship of the polar graphene oxide-mediated cellular response and stimulated growth of osteoblasts on hybrid chitosan network structure nanocomposite scaffolds. Acta Biomater. 2011;7(9):3432-3445.
[DOI] -
69. Qi J, Xiong Y, Cheng K, Huang Q, Cao J, He F, et al. Heterobifunctional PEG-grafted black phosphorus quantum dots: "Three-in-One" nano-platforms for mitochondria-targeted photothermal cancer therapy. Asian J Pharm Sci. 2021;16(2):222-235.
[DOI] -
70. Xu H, Fan M, Elhissi AMA, Zhang Z, Wan KW, Ahmed W, et al. PEGylated graphene oxide for tumor-targeted delivery of paclitaxel. Nanomedicine. 2015;10(8):1247-1262.
[DOI] -
71. Zheng Z, Cox M, Li B. Surface modification of hexagonal boron nitride nanomaterials: a review. J Mater Sci. 2018;53(1):66-99.
[DOI] -
72. Wang C, Chen B, Zou M, Cheng G. Cyclic RGD-modified chitosan/graphene oxide polymers for drug delivery and cellular imaging. Colloids Surf B Biointerfaces. 2014;122:332-340.
[DOI] -
73. Kanayama I, Miyaji H, Takita H, Nishida E, Tsuji M, Fugetsu B, et al. Comparative study of bioactivity of collagen scaffolds coated with graphene oxide and reduced graphene oxide. Int J Nanomedicine. 2014;9(1):3363-3373.
[DOI] -
74. Zhao H, Xing H, Lai Q, Zhao Y, Chen Q, Zou B. Additive manufacturing of graphene oxide/hydroxyapatite bioceramic scaffolds with reinforced osteoinductivity based on digital light processing technology. Mater Des. 2022;223:111231.
[DOI] -
75. Qi X, Liu Y, Yin X, Zhao R, Zhang W, Cao J, et al. Surface-based modified 3D-printed BG/GO scaffolds promote bone defect repair through bone immunomodulation. Compos Part B Eng. 2023;257:110673.
[DOI] -
76. Park J, Park S, Kim JE, Jang KJ, Seonwoo H, Chung J. Enhanced osteogenic differentiation of periodontal ligament stem cells using a graphene oxide-coated Poly(ε-caprolactone) scaffold. Polymers. 2021;13(5):797.
[DOI] -
77. Feng P, Shen S, Shuai Y, Peng S, Shuai C, Chen S. PLLA grafting draws GO from PGA phase to the interface in PLLA/PGA bone scaffold owing enhanced interfacial interaction. Sustain Mater Technol. 2023;35:e00566.
[DOI] -
78. Mahdavi R, Belgheisi G, Haghbin-Nazarpak M, Omidi M, Khojasteh A, Solati-Hashjin M. Bone tissue engineering gelatin-hydroxyapatite/graphene oxide scaffolds with the ability to release vitamin D: fabrication, characterization, and in vitro study. J Mater Sci: Mater Med. 2020;31:97.
[DOI] -
79. Sahafnejad-Mohammadi I, Rahmati S, Najmoddin N, Bodaghi M. Biomimetic polycaprolactone-graphene oxide composites for 3D printing bone scaffolds. Macromol Mater Eng. 2023;308(5):2200558.
[DOI] -
80. Wang G, Wei Z, Sang L, Chen G, Zhang W, Dong X, et al. Morphology, crystallization and mechanical properties of poly(ε-caprolactone)/graphene oxide nanocomposites. Chin J Polym Sci. 2013;31(8):1148-1160.
[DOI] -
81. Bow AJ, Masi TJ, Dhar MS. Etched 3D-printed polycaprolactone constructs functionalized with reduced graphene oxide for enhanced attachment of dental pulp-derived stem cells. Pharmaceutics. 2021;13(12):2146.
[DOI] -
82. Sharma A, Gupta S, Sampathkumar TS, Verma RS. Modified graphene oxide nanoplates reinforced 3D printed multifunctional scaffold for bone tissue engineering. Biomater Adv. 2022;134:112587.
[DOI] -
83. Alazab MH, Abouelgeit SA, Aboushelib MN. Histomorphometric evaluation of 3D printed graphene oxide-enriched poly(ε-caprolactone) scaffolds for bone regeneration. Heliyon. 2023;9(5):e15844.
[DOI] -
84. Guo W, Yang Y, Liu C, Bu W, Guo F, Li J, et al. 3D printed TPMS structural PLA/GO scaffold: Process parameter optimization, porous structure, mechanical and biological properties. J Mech Behav Biomed Mater. 2023;142:105848.
[DOI] -
85. Oladapo BI, Ismail SO, Adebiyi AV, Omigbodun FT, Olawumi MA, Olawade DB. Nanostructural interface and strength of polymer composite scaffolds applied to intervertebral bone. Colloids Surf A Physicochem Eng Asp. 2021;627:127190.
[DOI] -
86. Oladapo BI, Zahedi SA. Improving bioactivity and strength of PEEK composite polymer for bone application. Mater Chem Phys. 2021;266:124485.
[DOI] -
87. Oladapo BI, Zahedi SA, Ismail SO. Mechanical performances of hip implant design and fabrication with PEEK composite. Polymer. 2021;227:123865.
[DOI] -
88. Lu YC, Chang TK, Yeh ST, Lin TC, Lin HS, Chen CH, et al. Evaluation of graphene-derived bone scaffold exposure to the calvarial bone_in-vitro and in-vivo studies. Nanotoxicology. 2022;16(1):1-15.
[DOI] -
89. Li Y, He J, Zhou J, Li Z, Liu L, Hu S, et al. A conductive photothermal non-swelling nanocomposite hydrogel patch accelerating bone defect repair. Biomater Sci. 2022;10(5):1326-1341.
[DOI] -
90. Suo L, Xue Z, Wang P, Wu H, Chen Y, Shen J. Improvement of osteogenic properties using a 3D-printed graphene oxide/hyaluronic acid/chitosan composite scaffold. J Bioact Compat Polym. 2022;37(4):267-283.
[DOI] -
91. Ding P, Okoro OV, Sun Y, Wang L, Wei X, Liu S, et al. Graphene oxide-reinforced alginate/gelatin hydrogel via Schiff-base bond and thiol-Michael addition for bone regeneration. Mater Today Commun. 2022;33:104904.
[DOI] -
92. Li Y, Huang L, Tai G, Yan F, Cai L, Xin C, et al. Graphene Oxide-loaded magnetic nanoparticles within 3D hydrogel form High-performance scaffolds for bone regeneration and tumour treatment. Compos Part A Appl Sci Manuf. 2022;152:106672.
[DOI] -
93. Wang B, Yuan S, Xin W, Chen Y, Fu Q, Li L, et al. Synergic adhesive chemistry-based fabrication of BMP-2 immobilized silk fibroin hydrogel functionalized with hybrid nanomaterial to augment osteogenic differentiation of rBMSCs for bone defect repair. Int J Biol Macromol. 2021;192:407-416.
[DOI] -
94. Zhu S, Yao L, Pan C, Tian J, Li L, Luo B, et al. 3D printed gellan gum/graphene oxide scaffold for tumor therapy and bone reconstruction. Compos Sci Technol. 2021;208:108763.
[DOI] -
95. Zhang W, Chang Q, Xu L, Li G, Yang G, Ding X, et al. Graphene oxide-copper nanocomposite-coated porous CaP scaffold for vascularized bone regeneration via activation of hif-1α. Adv Healthc Mater. 2016;5(11):1299-1309.
[DOI] -
96. Kiumarsi N, Najmoddin N. Systematically engineered GO with magnetic CuFe2O4 to enhance bone regeneration on 3D printed PCL scaffold. Surfaces Interfaces. 2023;39:102973.
[DOI] -
97. Khan MUA, Al-Arjan WS, Ashammakhi N, Haider S, Amin R, Hasan A. Multifunctional bioactive scaffolds from ARX-g-(Zn@rGO)-HAp for bone tissue engineering: in vitro antibacterial, antitumor, and biocompatibility evaluations. Acs Applied Bio Mater. 2022;5(11):5445-5456.
[DOI] -
98. Li H, Shuai X, Chen Y, Xiong J, Zou Z, Peng S, et al. Engineering a wirelessly self-powered neural scaffold based on primary battery principle to accelerate nerve cell differentiation. Colloids Surf B Biointerfaces. 2025;249:114521.
[DOI] -
99. Fani K, Farahpour MR, Tabatabaei ZG. SeO32-/graphene oxide hybridized to multicomponent biopolymer based the scaffold to accelerate bone defect regeneration. Ceram Int. 2022;48(24):37212-37222.
[DOI] -
100. Shadianlou F, Foorginejad A, Yaghoubinezhad Y. Hydrothermal synthesis of zirconia-based nanocomposite powder reinforced by graphene and its application for bone scaffold with 3D printing. Adv Powder Technol. 2022;33(2):103406.
[DOI] -
101. Yang Y, Li M, Zhou B, Jiang X, Zhang D, Luo H, et al. Novel therapeutic strategy for bacteria-contaminated bone defects: reconstruction with multi-biofunctional GO/Cu-incorporated 3D scaffolds. Adv Ther. 2022;5 (7):2200043.
[DOI] -
102. Liu X, Gaihre B, Park S, Li L, Dashtdar B, Potes MDA, et al. 3D-printed scaffolds with 2D hetero-nanostructures and immunomodulatory cytokines provide pro-healing microenvironment for enhanced bone regeneration. Bioact Mater. 2023;27:216-230.
[DOI] -
103. Murugan E, Akshata CR. Graphene oxide reinforced SrHAP composite as a drug carrier in bone regeneration. Colloids Surf B Biointerfaces. 2022;219:112822.
[DOI] -
104. Zhao Y, Qiu Y, Fang Z, Pu F, Sun R, Chen K, et al. Preparation and characterization of Sr-substituted hydroxyapatite/reduced graphene oxide 3D scaffold as drug carrier for alendronate sodium delivery. Ceram Int. 2022;48(24):36601-36608.
[DOI] -
105. Zhang Y, Wang H, Huangfu H, Zhang X, Zhang H, Qin Q, et al. 3D printing of bone scaffolds for treating infected mandible bone defects through adjustable dual-release of chlorhexidine and osteogenic peptide. Mater Des. 2022;224:111288.
[DOI] -
106. Lee TH, Kim SY, Jang HW. Black phosphorus: critical review and potential for water splitting photocatalyst. Nanomaterials. 2016;6(11):194.
[DOI] -
107. Raucci MG, Fasolino I, Caporali M, Serrano-Ruiz M, Soriente A, Peruzzini M, et al. Exfoliated black phosphorus promotes in vitro bone regeneration and suppresses osteosarcoma progression through cancer-related inflammation inhibition. ACS Appl Mater Interfaces. 2019;11(9):9333-9342.
[DOI] -
108. Wang Y, Xie J, Kang J, Choi W, Jangili P, Zhang B, et al. Smart acid-activatable self-assembly of black phosphorous as photosensitizer to overcome poor tumor retention in photothermal therapy. Adv Funct Mater. 2020;30(42):2003338.
[DOI] -
109. Wu Y, Liao Q, Wu L, Luo Y, Zhang W, Guan M, et al. ZnL2-BPs integrated bone scaffold under sequential photothermal mediation: a win-win strategy delivering antibacterial therapy and fostering osteogenesis thereafter. Acs Nano. 2021;15(11):17854-17869.
[DOI] -
110. Deng F, Wu P, Qian G, Shuai Y, Zhang L, Peng S, et al. Silver-decorated black phosphorus: a synergistic antibacterial strategy. Nanotechnology. 2022;33(24):245708.
[DOI] -
111. Zhao Y, Peng X, Wang D, Zhang H, Xin Q, Wu M, et al. Chloroplast-inspired scaffold for infected bone defect therapy: towards stable photothermal properties and self-defensive functionality. Adv Sci. 2022;9(31):2204535.
[DOI] -
112. Li Z, Zhang X, Ouyang J, Chu D, Han F, Shi L, et al. Ca2+-supplying black phosphorus-based scaffolds fabricated with microfluidic technology for osteogenesis. Bioact Mater. 2021;6(11):4053-4064.
[DOI] -
113. Wang G, Qian G, Yao J, Cai W, Peng S, Shuai C. Polydopamine-decorated black phosphorous to enhance stability in polymer scaffold. Nanotechnology. 2021;32(45):455701.
[DOI] -
114. He M, Zhu C, Sun D, Liu Z, Du M, Huang Y, et al. Layer-by-layer assembled black phosphorus/chitosan composite coating for multi-functional PEEK bone scaffold. Compos Part B Eng. 2022;246:110266.
[DOI] -
115. Li W, Li S, Zhang J, Zhong H, Liang J, Huang S, et al. Fabrication and evaluation of bone morphogenetic protein-2 microspheres coated black phosphorus nanosheets@polylactic-glycolic acid copolymers scaffold: A multifunctional antibacterial photothermal scaffold for bone regeneration. Int J Biol Macromol. 2022;210:350-364.
[DOI] -
116. Xu D, Gan K, Wang Y, Wu Z, Wang Y, Zhang S, et al. A composite deferoxamine/black phosphorus nanosheet/gelatin hydrogel scaffold for ischemic tibial bone repair. Int J Nanomedicine. 2022;17:1015-1030.
[DOI] -
117. Miao Y, Chen Y, Luo J, Liu X, Yang Q, Shi X, et al. Black phosphorus nanosheets-enabled DNA hydrogel integrating 3D-printed scaffold for promoting vascularized bone regeneration. Bioact Mater. 2023;21:97-109.
[DOI] -
118. Wang X, Yu Y, Yang C, Shao C, Shi K, Shang L, et al. Microfluidic 3D printing responsive scaffolds with biomimetic enrichment channels for bone regeneration. Adv Funct Mater. 2021;31(40):2105190.
[DOI] -
119. Wang QH, Kalantar-Zadeh K, Kis A, Coleman JN, Strano MS. Electronics and optoelectronics of two-dimensional transition metal dichalcogenides. Nat Nanotechnol. 2012;7(11):699-712.
[DOI] -
120. Shuai C, Pan G, Wang Z, He T, Shuai X, Zhong Q, et al. Bifunctional MoS2@Cu2O heterojunction within scaffold for dual-mode synergistic antibacterial effects. Appl Surf Sci. 2025;686:162154.
[DOI] -
121. Lu L, Wang H, Yang M, Wang L, Gan KF. Three-dimensional-printed MPBI@β-TCP scaffold promotes bone regeneration and impedes osteosarcoma under near-infrared laser irradiation. Faseb J. 2023;37(5):e22924.
[DOI] -
122. Dai W, Zheng Y, Li B, Yang F, Chen W, Li Y, et al. A 3D-printed orthopedic implant with dual-effect synergy based on MoS2 and hydroxyapatite nanoparticles for tumor therapy and bone regeneration. Colloids Surf B Biointerfaces. 2023;228:113384.
[DOI] -
123. Qi F, Gao X, Peng S, Yang W, Qian G, Yang S, et al. Polyaniline Protrusions on MoS2 Nanosheets for PVDF Scaffolds with Improved Electrical Stimulation. Acs Appl Nano Mater. 2021;4 (12):13955-13966.
[DOI] -
124. Feng P, Shen S, Yang L, Kong Y, Yang S, Shuai C. Vertical and uniform growth of MoS2 nanosheets on GO nanosheets for efficient mechanical reinforcement in polymer scaffold. Virt Phys Prototyp. 2023;18(1):e2115384.
[DOI] -
125. Zheng L, Zhong Y, He T, Peng S, Yang L. A codispersed nanosystem of silver-anchored MoS2 enhances antibacterial and antitumor properties of selective laser sintered scaffolds. Int J Bioprint. 2022;8(3):111-125.
[DOI] -
126. Geng B, Li P, Fang F, Shi W, Glowacki J, Pan D, et al. Antibacterial and osteogenic carbon quantum dots for regeneration of bone defects infected with multidrug-resistant bacteria. Carbon. 2021;184:375-385.
[DOI] -
127. Zhang X, Li Y, Dong X, Wang H, Chen B, Li R, et al. 3D-printed bioactive ceramic scaffolds with MoSe2 nanocrystals as photothermal agents for bone tumor therapy. RSC Adv. 2022;12(47):30588-30597.
[DOI] -
128. Ding L, Wei Y, Wang Y, Chen H, Caro J, Wang H. A two-dimensional lamellar membrane: MXene nanosheet stacks. Angew Chem Int Ed Engl. 2017;56(7):1825-1829.
[DOI] -
129. Xue Q, Zhang H, Zhu M, Pei Z, Li H, Wang Z, et al. Photoluminescent Ti3C2 MXene quantum dots for multicolor cellular imaging. Adv Mater. 2017;29(15):1604847.
[DOI] -
130. Mi X, Su Z, Fu Y, Li S, Mo A. 3D printing of Ti3C2-MXene-incorporated composite scaffolds for accelerated bone regeneration. Biomed Mater. 2022;17(3):035002.
[DOI] -
131. Nie R, Sun Y, Lv H, Lu M, Huangfu H, Li Y, et al. 3D printing of MXene composite hydrogel scaffolds for photothermal antibacterial activity and bone regeneration in infected bone defect models. Nanoscale. 2022;14(22):8112-8129.
[DOI] -
132. Li F, Yan Y, Wang Y, Fan Y, Zou H, Liu H, et al. A bifunctional MXene-modified scaffold for photothermal therapy and maxillofacial tissue regeneration. Regen Biomater. 2021;8(6):rbab057.
[DOI] -
133. Xu Z, Zhang Y, Dai H, Wang Y, Ma Y, Tan S, et al. 3D printed MXene (Ti2AlN)/polycaprolactone composite scaffolds for in situ maxillofacial bone defect repair. J Ind Eng Chem. 2022;114:536-548.
[DOI] -
134. Han X, Jing X, Yang D, Lin H, Wang Z, Ran H, et al. Therapeutic mesopore construction on 2D Nb2C MXenes for targeted and enhanced chemo-photothermal cancer therapy in NIR-II biowindow. Theranostics. 2018;8(16):4491-4508.
[DOI] -
135. Yin J, Pan S, Guo X, Gao Y, Zhu D, Yang Q, et al. Nb2C MXene-functionalized scaffolds enables osteosarcoma phototherapy and angiogenesis/osteogenesis of bone defects. Nano-Micro Lett. 2021;13:30.
[DOI] -
136. He C, Yu L, Yao H, Chen Y, Hao Y. Combinatorial photothermal 3D-printing scaffold and checkpoint blockade inhibits growth/metastasis of breast cancer to bone and accelerates osteogenesis. Adv Funct Mater. 2021;31(10):2006214.
[DOI] -
137. Yang Q, Yin H, Xu T, Zhu D, Yin J, Chen Y, et al. Engineering 2D mesoporous silica@MXene-integrated 3D-printing scaffolds for combinatory osteosarcoma therapy and NO-augmented bone regeneration. Small. 2020;16(14):1906814.
[DOI] -
138. Miao S, Zhou J, Liu B, Lei X, Wang T, Hao X, et al. A 3D bioprinted nano-laponite hydrogel construct promotes osteogenesis by activating PI3K/AKT signaling pathway. Mater Today Bio. 2022;16:100342.
[DOI] -
139. Li S, Li Z, Yang J, Ha Y, Zhou X, He C. Inhibition of sympathetic activation by delivering calcium channel blockers from a 3D printed scaffold to promote bone defect repair. Adv Healthc Mater. 2022;11(16):2200785.
[DOI] -
140. Cebe T, Ahuja N, Monte F, Awad K, Vyavhare K, Aswath P, et al. Novel 3D-printed methacrylated chitosan-laponite nanosilicate composite scaffolds enhance cell growth and biomineral formation in MC3T3 pre-osteoblasts. J Mater Res. 2020;35:58-75.
[DOI] -
141. Xu X, Xiao L, Xu Y, Zhuo J, Yang X, Li L, et al. Vascularized bone regeneration accelerated by 3D-printed nanosilicate-functionalized polycaprolactone scaffold. Regen Biomater. 2021;8(6):rbab061.
[DOI] -
142. Cao B, Lin J, Tan J, Li J, Ran Z, Deng L, et al. 3D-printed vascularized biofunctional scaffold for bone regeneration. Int J Bioprint. 2023;9(3):185-199.
[DOI] -
143. Xiang H, Dai X, Xu W, Li S, Yang X, Huang Z, et al. Cryogenic 3D printing of bifunctional silicate nanoclay incorporated scaffolds for promoted angiogenesis and bone regeneration. Mater Des. 2022;223:111220.
[DOI] -
144. Dong L, Bu Z, Xiong Y, Zhang H, Fang J, Hu H, et al. Facile extrusion 3D printing of gelatine methacrylate/Laponite nanocomposite hydrogel with high concentration nanoclay for bone tissue regeneration. Int J Biol Macromol. 2021;188:72-81.
[DOI] -
145. Qi F, Liu W, Chen Y, Ding F, Li W, Peng S, et al. Near-infrared light-activated nanosystem endows scaffold with controllable nitric oxide release for peripheral nerve regeneration. J Colloid Interface Sci. 2025;682:210-221.
[DOI] -
146. Shu C, Qin C, Chen L, Wang Y, Shi Z, Yu J, et al. Metal-organic framework functionalized bioceramic scaffolds with antioxidative activity for enhanced osteochondral regeneration. Adv Sci. 2023;10(13):2206875.
[DOI] -
147. Jiang Y, Pan X, Yao M, Han L, Zhang X, Jia Z, et al. Bioinspired adhesive and tumor microenvironment responsive nanoMOFs assembled 3D-printed scaffold for anti-tumor therapy and bone regeneration. Nano Today. 2021;39:101182.
[DOI] -
148. Wang W, Xiong Y, Zhao R, Li X, Jia W. A novel hierarchical biofunctionalized 3D-printed porous Ti6Al4V scaffold with enhanced osteoporotic osseointegration through osteoimmunomodulation. J Nanobiotechnol. 2022;20:68.
[DOI] -
149. Jing C, Dong B, Zhang Y. Chemical modifications of layered double hydroxides in the supercapacitor. Energy Environ Mater. 2020;3(3):346-379.
[DOI] -
150. Fayyazbakhsh F, Solati-Hashjin M, Keshtkar A, Shokrgozar MA, Dehghan MM, Larijani B. Novel layered double hydroxides-hydroxyapatite/gelatin bone tissue engineering scaffolds: Fabrication, characterization, and in vivo study. Mater Sci Eng C. 2017;76:701-714.
[DOI] -
151. Camara-Torres M, Duarte S, Sinha R, Egizabal A, Alvarez N, Bastianini M, et al. 3D additive manufactured composite scaffolds with antibiotic-loaded lamellar fillers for bone infection prevention and tissue regeneration. Bioact Mater. 2021;6(4):1073-1082.
[DOI] -
152. Izbudak B, Bal-Ozturk A. The effect of LDHs nanoparticles on the cellular behavior of stem cell-laden 3D-bioprinted scaffold. J Biomater Appl. 2022;37(1):48-54.
[DOI] -
153. Alarcin E, Izbudak B, Erarslan EY, Domingo S, Tutar R, Titi K, et al. Optimization of methacrylated gelatin /layered double hydroxides nanocomposite cell-laden hydrogel bioinks with high printability for 3D extrusion bioprinting. J Biomed Mater Res A. 2023;111(2):209-223.
[DOI] -
154. Wang K, Ruan J, Song H, Zhang J, Wo Y, Guo S, et al. Biocompatibility of graphene oxide. Nanoscale Res Lett. 2011;6:8.
[DOI] -
155. Island JO, Steele GA, van der Zant HSJ, Castellanos-Gomez A. Environmental instability of few-layer black phosphorus. 2D Mater. 2015;2:1.
[DOI] -
156. Hu D, Li H, Wang B, Ye Z, Lei W, Jia F, et al. Surface-adaptive gold nanoparticles with effective adherence and enhanced photothermal ablation of methicillin-resistant Staphylococcus aureus biofilm. Acs Nano. 2017;11(9):9330-9339.
[DOI] -
157. Gao X, Deng W, Tan J, Shuai X, Zan J, Ye T, et al. Tumor microenvironment-responsive thermoelectric scaffold for on-demand antitumor therapy. Mater Today Chem. 2025;43:102455.
[DOI] -
158. Tong L, Liao Q, Zhao Y, Huang H, Gao A, Zhang W, et al. Near-infrared light control of bone regeneration with biodegradable photothermal osteoimplant. Biomaterials. 2019;193:1-11.
[DOI]
Copyright
© The Author(s) 2024. This is an Open Access article licensed under a Creative Commons Attribution 4.0 International License (https://creativecommons.org/licenses/by/4.0/), which permits unrestricted use, sharing, adaptation, distribution and reproduction in any medium or format, for any purpose, even commercially, as long as you give appropriate credit to the original author(s) and the source, provide a link to the Creative Commons license, and indicate if changes were made.
Publisher's Note
Share And Cite