Abstract
Photoacoustic imaging (PAI) is a rapidly growing biomedical imaging technique that combines the advantages of optical and acoustic imaging. This technique utilizes pulsed laser light to generate acoustic waves in biological tissue, which are then detected and used to create high-resolution images of tissue structure and function. PAI has several advantages over other imaging modalities, including its ability to provide functional information about tissue properties such as blood oxygenation and blood flow, as well as its ability to image tissue at greater depths than many other techniques. However, there are also challenges associated with PAI, including the need for specialized equipment and concerns regarding the potential for tissue damage due to laser exposure. Despite these challenges, PAI is a promising technique with many potential applications in biomedical research and clinical practice, and ongoing research in this area is likely to yield further advances in the coming years.
Keywords
1. Introduction
Photoacoustic imaging (PAI) is an emerging biomedical imaging technique that has shown great promise in improving our ability to visualize tissue structures and functional processes (Figure 1). The first photoacoustic imaging experiment was carried out in 1880 by Alexander Graham Bell, who used a thin strip of selenium to detect the sound waves produced by a beam of sunlight[1]. However, it was not until the 1990s that the modern technique of photoacoustic microscopy (PAM) was developed. In 1995, Lihong Wang and his colleagues at the Texas A & M University reported the first photoacoustic image of a biological tissue, using a focused laser beam to generate acoustic waves in a tissue sample[2]. Here is a brief overview of the major developments in PAM over the years (Table 1).
Development | Description |
Early Discovery | Alexander Graham Bell discovers the photoacoustic effect, demonstrating sound generation by illuminating materials with light in 1880. |
First Demonstration | In 1991, Kruger et al. demonstrated the first photoacoustic imaging in biological tissue. |
Concept Advancement | The thermoelastic expansion was introduced in 1994, improving the understanding of the photoacoustic effect. |
Handheld Probe | Zhang et al. developed a handheld photoacoustic probe for imaging blood vessels in 2002, enhancing portability and ease of use. |
Molecular-Specific Imaging | Molecular-specific photoacoustic imaging using targeted contrast agents was introduced in 2005, enabling specific visualization of molecular markers. |
Integration with Ultrasound | PAT emerged in 2008, integrating photoacoustic and ultrasound imaging for improved tissue characterization. |
Multispectral Imaging | Advancements in multispectral and three-dimensional photoacoustic imaging occurred around 2010, enabling enhanced tissue visualization and characterization. |
Real-Time Imaging | In 2013, real-time photoacoustic imaging systems were developed for clinical applications, providing immediate visualization during procedures. |
Functional Imaging | Functional photoacoustic imaging techniques, such as oxygen saturation imaging and blood flow imaging, were introduced in 2015, enabling the assessment of tissue function. |
Integration with Other Modalities | Integration of photoacoustic imaging with fluorescence imaging and MRI occurred in 2018, offering complementary imaging information. |
Miniaturization | In 2020, the miniaturization of photoacoustic imaging devices enabled endoscopic and intraoperative applications, expanding its clinical utility. |
Deep Learning Advancements | Recent advancements in deep learning algorithms for image reconstruction and analysis in photoacoustic imaging were made in 2022, improving image quality and analysis capabilities. |
PAT: photoacoustic tomography; MRI: magnetic resonance imaging.
2000s: Advances in laser technology, ultrasound transducers, and data processing algorithms led to significant improvements in PAM resolution and sensitivity. In 2003, Wang and his team developed a method for using PAM to image the microvasculature of biological tissues. This technique, called photoacoustic microscopy of microcirculation (PAMM), has been used to study blood flow in the brain, skin, and other tissues.
2010s: PAM has continued to evolve, with the development of new imaging modalities, such as functional PAM and photoacoustic computed tomography (PACT), which can provide three-dimensional images of biological tissues[3,4]. In 2013, Wang and his team developed a dual-wavelength PAM system that can distinguish between oxygenated and deoxygenated hemoglobin in blood vessels[5].
2020s: PAM is now widely used in biomedical research and clinical practice, with applications ranging from cancer diagnosis to brain imaging to monitoring of vascular disease. Researchers are also exploring new applications of PAM, such as the imaging of drug delivery and the detection of neural activity in the brain[6].
PAM has undergone significant development, with advances in laser technology, ultrasound transducers, and data processing algorithms[7,8]. In 2003, Wang and his team developed a method for using PAM to image the microvasculature of biological tissues[9,10]. This technique, called PAMM, has been used to study blood flow in the brain, skin, and other tissues. The technique works by illuminating tissue with a pulsed laser, which causes the tissue to heat up and expand, generating an acoustic wave that can be detected and used to create images.
Nguyen et al. reported Ultra-widefield photoacoustic microscopy with a dual-channel slider-crank laser-scanning apparatus for in vivo biomedical study[11]. The developed PAM system achieves an ultra-widefield scanning area of 24 mm and a high B-scan speed of 32 Hz, providing stable imaging. The spatial resolution of the system is measured to be approximately 3.4 μm laterally and 37 μm axially, indicating its ability to capture fine anatomical details such as BALB/c nude mouse ear (Figure 2i). One of the main advantages of PAI is its ability to provide high-resolution images of tissue structures at depths that are difficult to reach with other imaging modalities such as ultrasound or magnetic resonance imaging (MRI). This is due to the fact that acoustic waves can penetrate deep into tissue without being scattered or absorbed to a significant extent, allowing for highly detailed images to be obtained. Another advantage of PAI is its ability to provide functional information about tissue properties such as blood oxygenation and blood flow[12]. The strong PA signal from the skin layer often obscures the visualization of subcutaneous blood vessels, necessitating the development of methods to indirectly reconstruct PA images in human studies. To address this challenge, Ly et al. reported a deep learning (DL) model based on a modified U-Net architecture[13]. The DL model is designed to extract the relationship features between the amplitudes of the generated PA signal from the skin and the underlying blood vessels (Figure 2ii). By leveraging this DL-infused solution, the researchers aim to achieve high-resolution and high-contrast segmentation, enabling broader applications of PA imaging. By using different wavelengths of light to excite different types of tissue, it is possible to create images that reveal the distribution of oxygenated and deoxygenated blood, as well as the flow of blood through vessels[14].
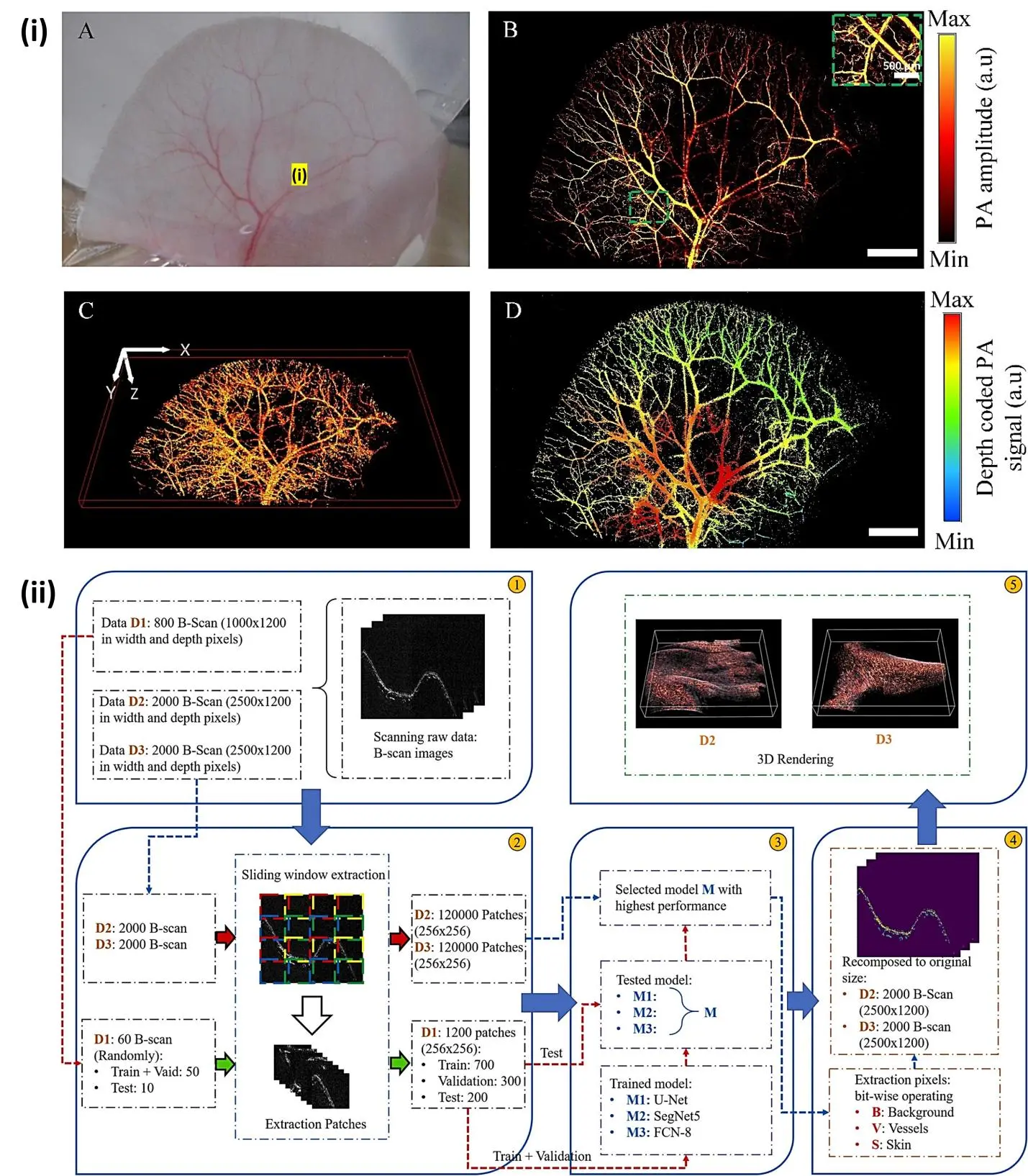
Lin et al. developed a three-dimensional photoacoustic computed tomography (3D-PACT) system to address the limitations of existing PACT systems[4]. This system offers a large imaging depth, scalable field of view, high imaging speed, and superior image quality (Figure 3). With 3D-PACT, detailed angiographic information can be obtained in various biological tissues, including the rat brain and the human breast. In the rat brain, the whole brain vasculatures and hemodynamics can be visualized, while in the human breast, an imaging depth of 4 cm can be achieved with a single breath hold of 10 s. The 3D-PACT system is a valuable tool for preclinical research and holds promise for clinical translation.
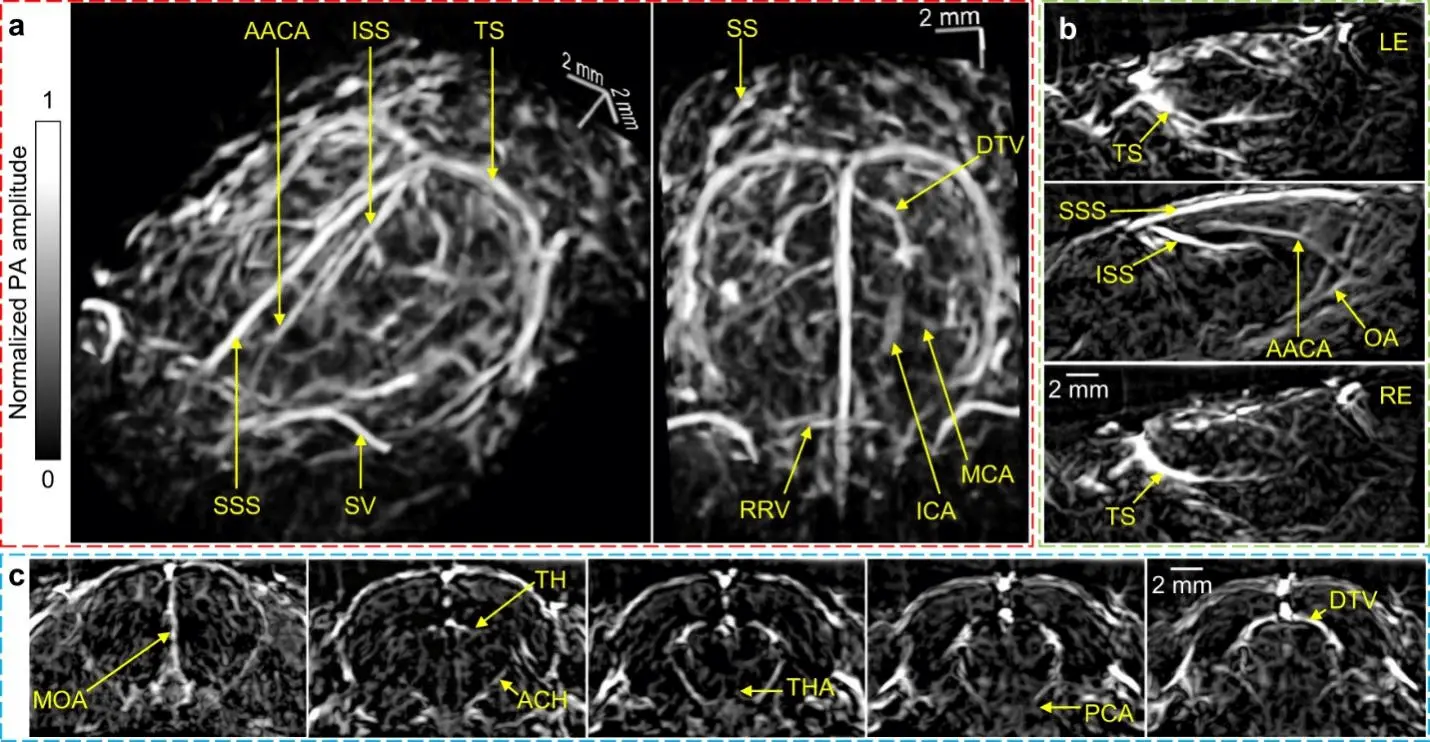
Figure 3. (a) Perspective angiograms of the rat's whole brain; (b) cross-sectional images of the brain on different transverse planes; (c) cross-sectional images of the brain on different coronal planes[4]. AACA: azygos of the anterior cerebral artery; ACH: anterior choroidal artery/vein; DTV: dorsal thalamic vein; ICA: internal carotid artery; ISS: inferior sagittal sinus; LE: left eye; MCA: middle cerebral artery; MOA: medial orbitofrontal artery; OA: olfactory artery; PCA: posterior cerebral artery; RE: right eye; RRV: rostral rhinal vein; SS: sigmoid sinus; SSS: superior sagittal sinus; SV: supraorbital vein; TH: transverse hippocampal artery/vein; THA: thalamoperforating artery; TS: transverse sinus.
Wong et al. demonstrated microtomy-assisted photoacoustic microscopy (mPAM), a technique that overcomes the challenge of three-dimensional (3D) optical imaging of biological organs with microscopic precision[15]. Without the need for tissue staining or clearing, mPAM automatically captures distortion-free and registration-free images, revealing endogenous absorption contrasts. By simultaneously sensing DNA/RNA, hemoglobins, and lipids, mPAM generates high-fidelity 3D images of whole organs embedded in paraffin or agarose, resembling histological imaging (Figure 4). With its deep tissue imaging capability and minimal sectioning artifacts, mPAM enables detailed analysis of cell nuclei, blood vessels, axons, and other anatomical structures, offering new insights into complex biological organs.
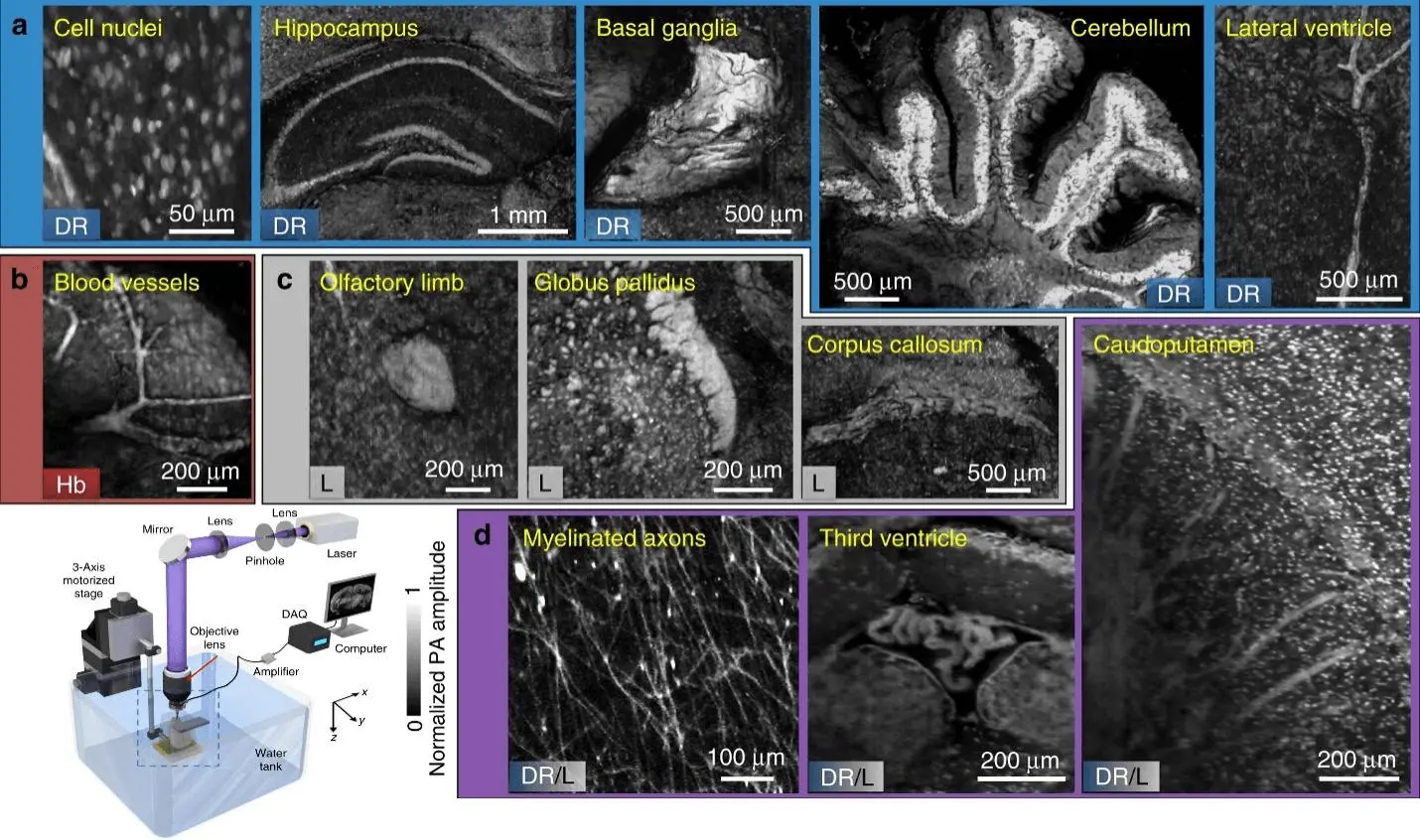
Figure 4. Images displaying characteristics obtained from label-free mPAM images of four unstained mouse brains preserved in agarose blocks are presented. All the features are depicted in a coronal view. The image set includes visuals highlighting the biomolecules responsible for absorption contrast, specifically DNA/RNA, hemoglobin, and lipids. Additionally, there are images showcasing myelinated axons, the third ventricle, and the caudoputamen, where the contrasts arise from both DNA/RNA and lipids. Schematic of the mPAM system for whole-organ imaging and sectioning (left down corner)[15]. mPAM: microtomy-assisted photoacoustic microscopy.
Despite these advantages, there are also some limitations to PAI must be addressed for it to become a widely used imaging technique. One of the main challenges is the need for specialized equipment, including high-powered lasers and sensitive acoustic detectors. This can make the technique expensive and difficult to implement in a clinical setting. Another limitation is the need for further research to fully understand the biological effects of laser exposure on tissue. While PAI is generally considered safe, there is still a need for more data on the potential long-term effects of repeated laser exposure.
2. Working Principle of Photoacoustic Imaging
Photoacoustic imaging is a non-invasive imaging technique that uses the photoacoustic effect to visualize biological tissue with high spatial resolution and excellent contrast[14]. The process of performing photoacoustic imaging can be broken down into the following steps[16,17].
Laser illumination: The first step in performing photoacoustic imaging is to illuminate the biological tissue with a short-pulsed laser beam. The tissue absorbs the laser energy, causing a rapid expansion and contraction of the tissue due to the photoacoustic effect.
Acoustic wave generation: As the tissue rapidly expands and contracts, it generates acoustic waves that propagate through the tissue. These acoustic waves are typically in the range of a few megahertz to tens of megahertz.
Acoustic wave detection: The generated acoustic waves are detected by ultrasound transducers placed on the skin surface. The transducers convert the acoustic waves into electrical signals that are amplified and digitized for further processing.
Image reconstruction: The detected acoustic signals are processed using signal processing techniques to reconstruct a 3D image of the tissue. The photoacoustic signal is proportional to the optical absorption coefficient of the tissue, which provides information about the tissue morphology and function.
Photoacoustic imaging can be performed using various laser sources, including pulsed lasers and continuous wave lasers. The choice of laser source depends on the specific application and the desired imaging depth. Additionally, photoacoustic imaging can be performed in various modes, including linear and nonlinear modes, depending on the desired imaging resolution and contrast[11]. There have been several recent advances in photoacoustic biomedical imaging that have the potential to enhance the capabilities and applications of this technique. Here are some examples:
Molecular imaging: Researchers have developed targeted photoacoustic contrast agents that can be used to image specific molecular markers in tissues, such as cancer cells or inflammation markers. This enables molecular-level imaging with high specificity and sensitivity[18].
Deep tissue imaging: New approaches to PAI, such as multispectral optoacoustic tomography, have enabled imaging of tissue at depths beyond what was previously possible[19]. This has the potential to improve the diagnosis and monitoring of diseases in deeper tissues.
Real-time imaging: Advances in image acquisition and processing have enabled real-time photoacoustic imaging, which can provide dynamic information about tissue function and response to therapy[20].
3D imaging: New techniques for 3D photoacoustic imaging, such as photoacoustic holography, have been developed, enabling high-resolution 3D imaging of tissue structures and functions.
Clinical translation: There has been increasing interest in translating PAI into clinical practice, with several studies demonstrating its potential for non-invasive diagnosis and monitoring of diseases such as cancer, cardiovascular disease, and neurological disorders[13].
Recent advances in photoacoustic biomedical imaging have the potential to transform the way we diagnose and monitor diseases. As research in this area continues, we can expect to see further developments and refinements to these techniques, as well as new applications in areas such as drug development and personalized medicine. PAI is a promising technique that has the potential to revolutionize biomedical imaging by providing high-resolution images of tissue structures and functional processes. As research in this area continues, we will likely to see the development of new and improved PAI systems that are more affordable and easier to use, making this technique more accessible to researchers and clinicians alike.
3. Role of Contrast Agent in Photoacoustic Imaging
Contrast agents can be used in photoacoustic imaging to enhance the contrast between different tissues or to improve the sensitivity and specificity of the imaging modality. Various contrast agents can be used in photoacoustic imaging, including exogenous contrast agents and endogenous contrast agents[21] (Table 2). Exogenous contrast agents are typically administered prior to imaging and are designed to selectively accumulate in the tissue of interest, thereby enhancing the photoacoustic signal. These agents can be divided into two categories: molecular contrast agents and nanoparticle-based contrast agents. Molecular contrast agents are small molecules that can selectively bind to specific targets in the tissue[37]. For example, some molecular contrast agents can selectively bind to cancer cells or angiogenic blood vessels, which are commonly found in tumors. These agents can be designed to emit photoacoustic signals when they bind to their targets, providing high specificity for the tissue of interest. Nanoparticle-based contrast agents are nanoparticles that can be designed to accumulate in the tissue of interest, thereby enhancing the photoacoustic signal[38]. These nanoparticles can be functionalized with specific ligands or antibodies to target specific cells or tissues. For example, gold nanoparticles can be used as contrast agents in photoacoustic imaging due to their strong photoacoustic signal and biocompatibility[39]. Silver nanoparticles have been investigated as a potential photoacoustic contrast agent due to their strong optical absorption in the visible and near-infrared regions of the electromagnetic spectrum[40]. Iron oxide nanoparticles are another type of nanoparticle that can be used as contrast agents in photoacoustic imaging[41]. They have high magnetic susceptibility, which allows them to generate a strong photoacoustic signal under a magnetic field. Carbon-based nanoparticles, such as carbon nanotubes and graphene oxide, have also been investigated as contrast agents for photoacoustic imaging[42]. They have strong optical absorption in the near-infrared region and can be synthesized in various sizes and shapes to optimize their photoacoustic properties. Endogenous contrast agents are naturally occurring molecules in the tissue that can generate photoacoustic signals. These agents include hemoglobin, which can generate a strong photoacoustic signal due to its high optical absorption, and melanin, which can generate a photoacoustic signal in skin tissue[43]. The choice of contrast agent depends on the specific application and the desired imaging contrast and sensitivity. The use of contrast agents in photoacoustic imaging has the potential to improve the accuracy and specificity of the imaging modality, making it a promising tool for a wide range of clinical applications.
Imaging Modalities | Contrast agent | Excitation (nm) | Concentration | Tracking days | Application |
PAI | ICG | 810 | 0.25 mg/ml | 3 days | Cell transplantation therapies[22] |
PAI | AuNS | 700 | 1.5 ng gold/cell | -- | Neuro degenerative diseases[23] |
PAI | 700 | 200 μg gold/ml | -- | Glaucoma treatment[24] | |
PAI | Gold Nano Rods | 680 | 1.5×106 SiGNRs/cell | 4 days | Muscular dystrophy[25] |
PAI | 760 | 3.5×10-2 μg Au/ml | 15 days | In vivo stem cell tracking[26] | |
PAI | 790,920 | 20 μg/ml | 10 days | Stem cell viability tracking[27] | |
PAI, MRI | SPIO@Au | 810 | 4 μg/ml | 3 days | Brain lesion treatment[28] |
PAI, Two-photon microscopy | AuNC | 800 | 2.3×104 AuNCs/cell | Tracking cells[29] | |
PAI | IO | 532,700 | 20 μg/ml | -- | Delivery of resveratrol[30] |
US, PAI, MPI | IO nanobubbles labelled with DiR | 240 μg/ml | 3 days | Cardiac therapy[31] | |
PAI, MRI | Fe3+-PEG-MNP | 800 μg/ml | 35 days | Tracking cells[32] | |
PAI | Prussian blue particles | 701 | 0.2 mg/ml | 15 days | Brain injury treatment[33] |
PAI | Prussian blue nanoparticles | 730 | 50 μg/ml | 14 days | Ischemic brain damage[34] |
PAI | Organic semiconducting polymer nanoparticle probes + | 916,1,025 | 50 μg/ ml | 14 days | Tracking cells[35] |
PAI, MRI | gadolinium loadedmelanin nanoparticles | 720-760 | 0.43 mg/ml | -- | In vivo stem cell tracking[36] |
PAI: photoacoustic imaging; ICG: Indocyanine Green; MRI: magnetic resonance imaging; IO: iron oxide; AuNS: Gold Nanostructures; US: Ultrasound Imaging.
4. The Future of Photoacoustic Imaging
The future of photoacoustic imaging (PAI) looks bright as this emerging technique continues to show great promise for biomedical imaging applications. Here are some potential developments that could shape the future of PAI. Improved sensitivity and resolution: Advances in laser and detector technology could lead to PAI systems with improved sensitivity and resolution, enabling even higher-quality images to be obtained. Multimodal imaging: Combining PAI with other imaging modalities such as MRI or CT could provide even more comprehensive information about tissue structure and function. Photoacoustic computed tomography (PACT) is a noninvasive hybrid imaging technique that combines the advantages of optical and ultrasound imaging modalities. It enables the visualization of both structural and functional information in biological tissues. Clinical translation: While PAI is still primarily a research tool, there is great potential for it to be used in a clinical setting for applications such as cancer detection and monitoring. As the technology becomes more affordable and easier to use, we may see more widespread adoption of PAI in clinical practice. Therapeutic applications : PAI could also be used in conjunction with other therapies such as photothermal or photodynamic therapy, which use light to destroy cancer cells or other diseased tissue. Wearable devices : Miniaturized PAI systems could be integrated into wearable devices for continuous monitoring of physiological parameters such as blood flow or oxygenation.
5. VOSviewer Bibliometric Analysis of Photoacoustic Imaging
VOSviewer is a software tool that can be used for analyzing bibliometric data, such as data related to scientific publications[44]. VOSviewer is a software tool used for analyzing bibliometric data related to scientific publications. It employs a network visualization approach to analyze relationships between items, such as publications or authors. In the context of photoacoustic imaging, VOSviewer can be utilized to examine bibliographic data on this subject. It performs various bibliometric analyses, including co-authorship analysis, citation analysis, and co-citation analysis. Co-authorship analysis identifies collaborations between authors based on shared publications, citation analysis identifies references made by one publication to another, and co-citation analysis identifies publications frequently cited together. After constructing the network and conducting the analysis, VOSviewer visualizes the network as a map, representing items as nodes and relationships as links. It also calculates metrics such as centrality measures, clustering coefficients, and modularity to describe the network.
When it comes to photoacoustic imaging, the utilization of VOSviewer offers the opportunity to create a network illustrating the connections between publications in this field. Additionally, it enables conducting co-citation analysis to identify the publications that are most frequently cited within the domain, as illustrated in Figure 5.
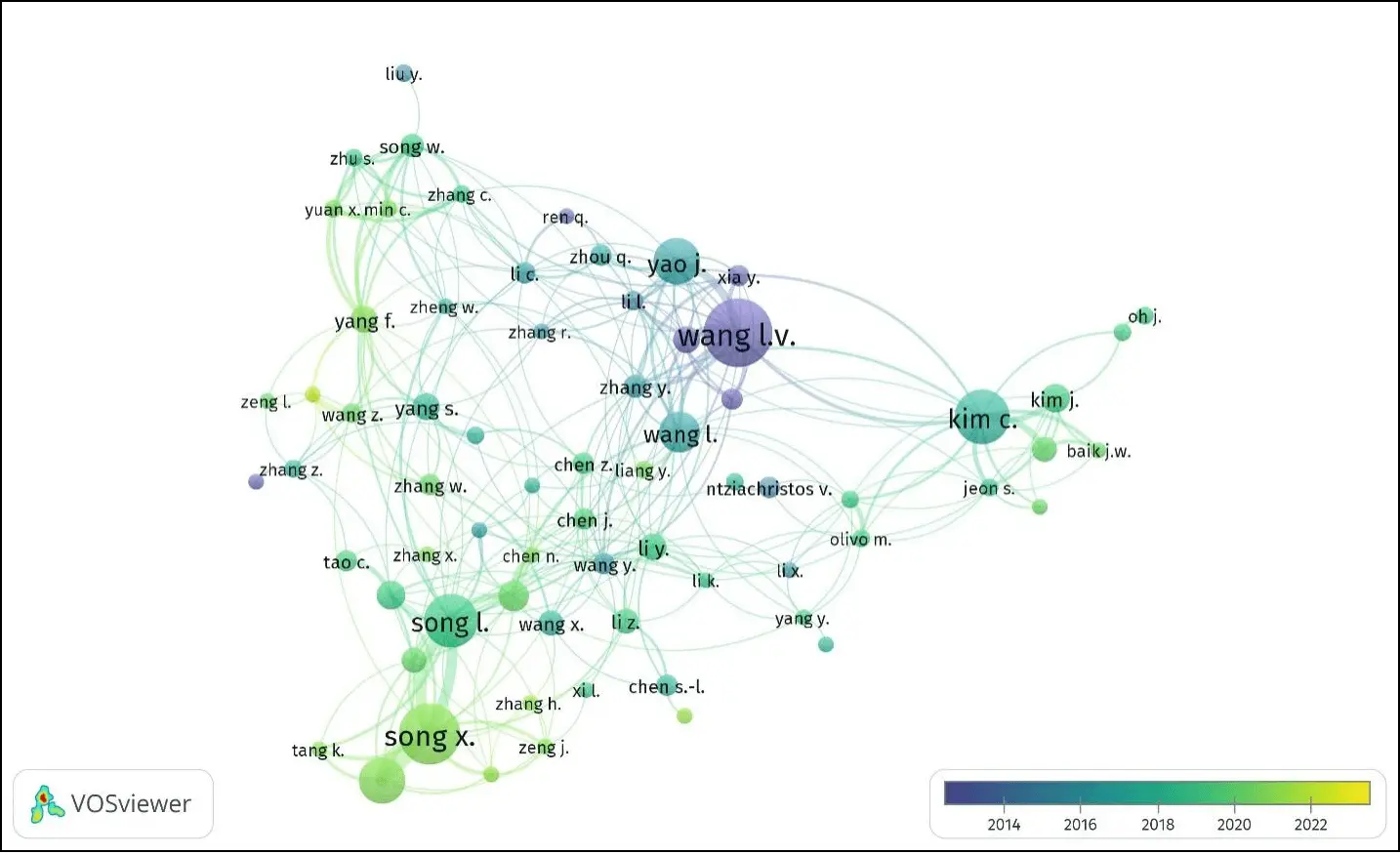
Figure 5. VOSviewer bibliometric data analysis of year-wise publications on photoacoustic imaging.
By utilizing this approach, valuable insights can be gained regarding the structure and advancement of the field of photoacoustic imaging. The generated map and the associated metrics derived from the analysis can provide a comprehensive understanding of the relationships and trends within the research literature. This allows researchers and practitioners to identify key works, influential authors, and emerging topics, ultimately fostering the development and progress of photoacoustic imaging techniques and applications. For this bibliometric study, data from the last 10 years (2013-2023) was analyzed to identify research groups with initial impactful research work. The color scale indicates the research publication and achievements from 2013 to 2023. The label size variation confirms the research contribution in the field of PAM. The curved lines indicate the research connections between each group. This type of bibliometric analysis helps to analyze and identify the contribution of each researcher in a single graph.
6. Limitations of Photoacoustic Biomedical Imaging
While PAI is a promising biomedical imaging technique, there are several limitations must be considered. Limited imaging depth: While PAI is capable of imaging deeper tissue layers than some other techniques, there remains a limit to the depth that can be imaged. This limit is determined by the optical and acoustic properties of the tissue, as well as the wavelength of the laser used. Tissue heterogeneity: Tissues within the body are not uniform in their optical and acoustic properties, which can lead to variations in the signal detected by the PAI system. This can make it difficult to obtain accurate and reliable images, particularly in complex tissue structures. Cost and complexity: PAI requires specialized equipment, including high-powered lasers and sensitive acoustic detectors, which can be expensive and difficult to maintain. This can limit the accessibility of PAI to many researchers and clinicians. Safety concerns: There is still some uncertainty about the long-term effects of repeated laser exposure on tissue, and there is a risk of tissue damage if the laser intensity is too high. This risk must be carefully balanced against the potential benefits of PAI. Limited functional information: While PAI is capable of measuring physiological parameters such as blood oxygenation and blood flow, these measurements are limited to the tissue volume that is directly imaged. This can make it difficult to obtain a comprehensive picture of tissue function.
7. Conclusion
Photoacoustic imaging is a cutting-edge technique that combines the strengths of optical and acoustic imaging to provide high-resolution and contrast images of biological tissues and organs. The technique is based on the generation of acoustic waves by the absorption of light in biological tissues, which can then be detected using ultrasound sensors. Since its first introduction in the early 1990s, photoacoustic imaging has shown great potential in various applications, including cancer detection, functional brain imaging, and cardiovascular imaging. One major benefit of photoacoustic imaging is its ability to provide high-resolution images of biological tissues with high contrast. This is because the absorption of light in biological tissues is highly specific to the type and concentration of the chromophores present, such as hemoglobin, melanin, and lipids. By tuning the wavelength of the excitation light, it is possible to selectively target different chromophores and obtain images with specific contrast, allowing for the visualization of different tissue types and functions. Another advantage of photoacoustic imaging is its ability to provide functional information, such as blood flow and oxygenation, in addition to anatomical information. This is because the absorption of light by hemoglobin is highly dependent on its oxygenation state, and by measuring the photoacoustic signals generated by hemoglobin, it is possible to obtain information about the blood flow and oxygenation of tissues. This is particularly useful in cancer detection and diagnosis, where changes in blood flow and oxygenation are often associated with the presence of tumors.
One of the major applications of photoacoustic imaging is in cancer detection and diagnosis. In cancer, there is often an increase in blood flow and changes in the oxygenation state of tissues, which can be detected using photoacoustic imaging. By selectively targeting the chromophores present in cancerous tissues, such as hemoglobin and melanin, it is possible to obtain high-resolution images of tumors with high contrast. This has the potential to improve cancer diagnosis and treatment by enabling early detection and precise targeting of tumors. Another promising application of photoacoustic imaging is in functional brain imaging. By measuring the photoacoustic signals generated by hemoglobin, it is possible to obtain information about the blood flow and oxygenation of brain tissues, which is highly correlated with brain activity. This technique has the potential to improve our understanding of brain function and enable the diagnosis and treatment of neurological disorders.
Finally, photoacoustic imaging has the potential to revolutionize cardiovascular imaging by providing high-resolution images of blood vessels and detecting early signs of cardiovascular disease. By selectively targeting the chromophores present in the blood, such as hemoglobin, it is possible to obtain high-contrast images of blood vessels and detect changes in blood flow and oxygenation that are associated with cardiovascular disease. Despite its many advantages, there are still some technical and practical challenges that need to be addressed to fully realize the potential of photoacoustic imaging. One of the major challenges is improving imaging depth, as the absorption of light in biological tissues decreases with increasing depth. This can be addressed by developing new contrast agents and improving the sensitivity of ultrasound sensors. Another challenge is developing new contrast agents that are specific to different tissue types and functions, such as cancer cells and neuronal activity.
In conclusion, photoacoustic imaging is a promising and emerging technique for biomedical imaging that has shown tremendous potential in various applications, including cancer detection, functional brain imaging, and cardiovascular imaging. The ability of this technique to combine the advantages of both optical and acoustic imaging makes it a powerful tool for various applications in clinical and preclinical research. Despite the challenges, photoacoustic imaging has great potential to revolutionize the field of biomedical imaging and create new avenues for research and diagnosis in various areas of medicine.
Authors contribution
Mondal S: Conceptualization, literature review, validation, writing original draft, interpreted the data.
Park S, Choi J: Literature review, image preparation, writing-review & editing.
Oh J: Conceptualization, writing-review & editing, funding acquisition, supervision.
All authors approved the final version of the manuscript.
Conflicts of interest
The authors declare no conflict of interest.
Ethical approval
Not applicable.
Consent to participate
Not applicable.
Consent for publication
Not applicable.
Availability of data and materials
Not applicable.
Funding
This work was supported by the National Research Foundation of Korea (NRF) grant funded by the Korea government (MSIT) (No. 2022R1A5A8023404).
Copyright
© The Author(s) 2023.
References
-
1. Su Y, Zhang F, Xu K, Yao J, Wang RK. A photoacoustic tomography system for imaging of biological tissues. J Phys D Appl Phys. 2005;38(15):2640-2644.
[DOI] -
2. Lungu GF, Li ML, Xie X, Wang LV, Stoica G. In vivo imaging and characterization of hypoxia-induced neovascularization and tumor invasion. Int J Oncol. 2007;30(1):45-54.
[PubMed] -
3. Wang LV, Gao L. Photoacoustic microscopy and computed tomography: from bench to bedside. Annu Rev Biomed Eng. 2014;16:155-185.
[DOI] [PubMed] [PMC] -
4. Lin L, Hu P, Tong X, Na S, Cao R, Yuan X, et al. High-speed three-dimensional photoacoustic computed tomography for preclinical research and clinical translation. Nat Commun. 2021;12(1):882.
[DOI] -
5. Hu S, Wang LV. Optical-resolution photoacoustic microscopy: auscultation of biological systems at the cellular level. Biophys J. 2013;105(4):841-847.
[DOI] [PubMed] [PMC] -
6. Yao J, Wang LV. Photoacoustic microscopy. Laser Photon Rev. 2013;7(5):758-778.
[DOI] [PubMed] [PMC] -
7. Das D, Sharma A, Rajendran P, Pramanik M. Another decade of photoacoustic imaging. Phys Med Biol. 2021;66(5):05TR01.
[DOI] [PubMed] -
8. Pham VH, Nguyen VT, Ly CD, Choi J, Park S, Mondal S, et al. Development of fast photoacoustic and ultrasound imaging system based on slider-crank scanner for small animals and humans study. Expert Syst Appl. 2022;206:117939.
[DOI] -
9. Kim C, Favazza C, Wang LV. In vivo photoacoustic tomography of chemicals: high-resolution functional and molecular optical imaging at new depths. Chem Rev. 2010;110(5):2756-2782.
[DOI] [PubMed] [PMC] -
10. Xie Z, Jiao S, Zhang HF, Puliafito CA. Laser-scanning optical-resolution photoacoustic microscopy. Opt Lett. 2009;34(12):1771-1773.
[DOI] [PubMed] -
11. Nguyen VT, Truong NTP, Pham VH, Choi J, Park S, Ly CD, et al. Ultra-widefield photoacoustic microscopy with a dual-channel slider-crank laser-scanning apparatus for in vivo biomedical study. Photoacoustics. 2021;23:100274.
[DOI] [PubMed] [PMC] -
12. Mallidi S, Luke GP, Emelianov S. Photoacoustic imaging in cancer detection, diagnosis, and treatment guidance. Trends Biotechnol. 2011;29(5):213-221.
[DOI] [PubMed] [PMC] -
13. Ly CD, Nguyen VT, Vo TH, Mondal S, Park S, Choi J, et al. Full-view in vivo skin and blood vessels profile segmentation in photoacoustic imaging based on deep learning. Photoacoustics. 2022;25:100310.
[DOI] -
14. Rajian JR, Carson PL, Wang X. Quantitative photoacoustic measurement of tissue optical absorption spectrum aided by an optical contrast agent. Opt Express. 2009;17(6):4879-4889.
[DOI] [PubMed] [PMC] -
15. Wong TTW, Zhang R, Zhang C, Hsu HC, Maslov KI, Wang L, et al. Label-free automated three-dimensional imaging of whole organs by microtomy-assisted photoacoustic microscopy. Nat Commun. 2017;8:1386.
[DOI] -
16. Xiang L, Wang B, Ji L, Jiang H. 4-D photoacoustic tomography. Sci Rep. 2013;3:1113.
[DOI] [PubMed] [PMC] -
17. Zhang Q, Liu Z, Carney PR, Yuan Z, Chen H, Roper SN, et al. Non-invasive imaging of epileptic seizures in vivo using photoacoustic tomography. Phys Med Biol. 2008;53(7):1921-1931.
[DOI] [PubMed] -
18. Li Y, Chen Y, Du M, Chen ZY. Ultrasound technology for molecular imaging: from contrast agents to multimodal imaging. ACS Biomater Sci Eng. 2018;4(8):2716-2728.
[DOI] [PubMed] -
19. Ntziachristos V, Razansky D. Molecular imaging by means of multispectral optoacoustic tomography (MSOT). Chem Rev. 2010;110(5):2783-2794.
[DOI] [PubMed] -
20. Lin L, Wang LV. The emerging role of photoacoustic imaging in clinical oncology. Nat Rev Clin Oncol. 2022;19(6):365-384.
[DOI] [PubMed] -
21. James S, Neuhaus K, Murphy M, Leahy M. Contrast agents for photoacoustic imaging: a review of stem cell tracking. Stem Cell Res Ther. 2021;12(1):511.
[DOI] [PubMed] [PMC] -
22. Filippi M, Garello F, Pasquino C, Arena F, Giustetto P, Antico F, et al. Indocyanine green labeling for optical and photoacoustic imaging of mesenchymal stem cells after in vivo transplantation. J Biophotonics. 2019;12(5):e201800035.
[DOI] [PubMed] -
23. Donnelly EM, Kubelick KP, Dumani DS, Emelianov SY. Photoacoustic image-guided delivery of plasmonic-nanoparticle-labeled mesenchymal stem cells to the spinal cord. Nano Lett. 2018;18(10):6625-6632.
[DOI] [PubMed] -
24. Kubelick KP, Snider EJ, Ethier CR, Emelianov S. Development of a stem cell tracking platform for ophthalmic applications using ultrasound and photoacoustic imaging. Theranostics. 2019;9(13):3812-3824.
[DOI] [PubMed] [PMC] -
25. Jokerst JV, Thangaraj M, Kempen PJ, Sinclair R, Gambhir SS. Photoacoustic imaging of mesenchymal stem cells in living mice via silica-coated gold nanorods. ACS Nano. 2012;6(7):5920-5930.
[DOI] [PubMed] [PMC] -
26. Comenge J, Fragueiro O, Sharkey J, Taylor A, Held M, Burton NC, et al. Preventing plasmon coupling between gold nanorods improves the sensitivity of photoacoustic detection of labeled stem cells in vivo. ACS Nano. 2016;10(7):7106-7116.
[DOI] [PubMed] -
27. Dhada KS, Hernandez DS, Suggs LJ. In vivo photoacoustic tracking of mesenchymal stem cell viability. ACS Nano. 2019;13(7):7791-7799.
[DOI] [PubMed] [PMC] -
28. Qiao Y, Gumin J, MacLellan CJ, Gao F, Bouchard R, Lang FF, et al. Magnetic resonance and photoacoustic imaging of brain tumor mediated by mesenchymal stem cell labeled with multifunctional nanoparticle introduced via carotid artery injection. Nanotechnology. 2018;29(16):165101.
[DOI] [PubMed] [PMC] -
29. Zhang YS, Wang Y, Wang L, Wang Y, Cai X, Zhang C, et al. Labeling human mesenchymal stem cells with gold nanocages for in vitro and in vivo tracking by two-photon microscopy and photoacoustic microscopy. Theranostics. 2013;3(8):532-543.
[DOI] [PubMed] [PMC] -
30. Adjei IM, Yang H, Plumton G, Maldonado-Camargo L, Dobson J, Rinaldi C, et al. Multifunctional nanoparticles for intracellular drug delivery and photoacoustic imaging of mesenchymal stem cells. Drug Deliv Transl Res. 2019;9(3):652-666.
[DOI] [PubMed] -
31. Lemaster JE, Chen F, Kim T, Hariri A, Jokerst JV. Development of a trimodal contrast agent for acoustic and magnetic particle imaging of stem cells. ACS Appl Nano Mater. 2018;1(3):1321-1331.
[DOI] [PubMed] [PMC] -
32. Zhang H, Wang ZJ, Wang LJ, Li TT, He S, Li LP, et al. A dual-mode nanoparticle based on natural biomaterials for photoacoustic and magnetic resonance imaging of bone mesenchymal stem cells in vivo. RSC Adv. 2019;9(60):35003-35010.
[DOI] [PubMed] [PMC] -
33. Li W, Chen R, Lv J, Wang H, Liu Y, Peng Y, et al. In vivo photoacoustic imaging of brain injury and rehabilitation by high-efficient near-infrared dye labeled mesenchymal stem cells with enhanced brain barrier permeability. Adv Sci. 2017;5(2):1700277.
[DOI] [PubMed] [PMC] -
34. Kim T, Lemaster JE, Chen F, Li J, Jokerst JV. Photoacoustic imaging of human mesenchymal stem cells labeled with Prussian Blue-Poly(L-lysine) nanocomplexes. ACS Nano. 2017;11(9):9022-9032.
[DOI] [PubMed] [PMC] -
35. Yin C, Wen G, Liu C, Yang B, Lin S, Huang J, et al. Organic semiconducting polymer nanoparticles for photoacoustic labeling and tracking of stem cells in the second near-infrared window. ACS Nano. 2018;12(12):12201-12211.
[DOI] [PubMed] -
36. Lemaster JE, Wang Z, Hariri A, Chen F, Hu Z, Huang Y, et al. Gadolinium doping enhances the photoacoustic signal of synthetic melanin nanoparticles: A dual modality contrast agent for stem cell imaging. Chem Mater. 2019;31(1):251-259.
[DOI] [PubMed] [PMC] -
37. Weber J, Beard PC, Bohndiek SE. Contrast agents for molecular photoacoustic imaging. Nat Methods. 2016;13(8):639-650.
[DOI] [PubMed] -
38. Lemaster JE, Jokerst JV. What is new in nanoparticle-based photoacoustic imaging? WIREs Nanomed Nanobiotechnol. 2017;9(1):e1404.
[DOI] [PubMed] [PMC] -
39. Cheheltani R, Ezzibdeh RM, Chhour P, Pulaparthi K, Kim J, Jurcova M, et al. Tunable, biodegradable gold nanoparticles as contrast agents for computed tomography and photoacoustic imaging. Biomaterials. 2016;102:87-97.
[DOI] [PubMed] [PMC] -
40. Mondal S, Montaño-Priede JL, Nguyen VT, Park S, Choi J, Doan VHM, et al. Computational analysis of drug free silver triangular nanoprism theranostic probe plasmonic behavior for in-situ tumor imaging and photothermal therapy. J Adv Res. 2022;41:23-38.
[DOI] [PubMed] [PMC] -
41. Xi L, Grobmyer SR, Zhou G, Qian W, Yang L, Jiang H. Molecular photoacoustic tomography of breast cancer using receptor targeted magnetic iron oxide nanoparticles as contrast agents. J Biophotonics. 2014;7(6):401-409.
[DOI] [PubMed] [PMC] -
42. Fu Q, Zhu R, Song J, Yang H, Chen X. Photoacoustic imaging: contrast agents and their biomedical applications. Adv Mater. 2019;31(6):e1805875.
[DOI] [PubMed] -
43. Upputuri PK, Pramanik M. Recent advances in photoacoustic contrast agents for in vivo imaging. Wiley Interdiscip Rev Nanomed Nanobiotechnol. 2020;12(4):e1618.
[DOI] [PubMed] -
44. Eck NJ, Waltman L. Software survey: VOSviewer, a computer program for bibliometric mapping. Scientometrics. 2010;84(2):523-538.
[DOI] [PubMed] [PMC]
Copyright
© The Author(s) 2023. This is an Open Access article licensed under a Creative Commons Attribution 4.0 International License (https://creativecommons.org/licenses/by/4.0/), which permits unrestricted use, sharing, adaptation, distribution and reproduction in any medium or format, for any purpose, even commercially, as long as you give appropriate credit to the original author(s) and the source, provide a link to the Creative Commons license, and indicate if changes were made.
Publisher’s Note
Share And Cite